Page snapshot: Introduction to the types of evidence used to study grasses in the paleontological (fossil) and archaeological records, including macroremains, pollen, phytoliths, starch grains, mammal teeth, and isotopes.
Topics covered on this page: Introduction; Macroremains; Pollen; Epidermis and phytoliths; Starch grains; Indirect evidence; Mammal tooth morphology; Carbon isotopes; Resources.
Credits: Funded by the National Science Foundation. Any opinions, findings, and conclusions or recommendations expressed in this material are those of the author(s) and do not necessarily reflect the views of the National Science Foundation. Page by Elizabeth J. Hermsen (2022).
Updates: Page last updated January 11, 2023.
Image above: Scanning electron micrographs (pictures taken with a scanning electron microscope) of elephant grass (Cenchrus purpureus) phytoliths. Left photo and right photo by Benjamin Gadet (Wikimedia Commons, Creative Commons Attribution-ShareAlike 3.0 Unported license, images cropped and resized).
Introduction
While paleontology and archaeology are often confused with one another, the two fields of study are distinct. In both fields, scientists use physical evidence to study the past. The difference is that paleontologists are primarily interested in the study of ancient life, whereas archaeologists focus on the study of human culture.
Paleobotany is the subdivision of paleontology that deals with the study of plant fossils, including the structure, evolution, and ecology of ancient plants; archaeobotany or paleoethnobotany is the study of plants and their uses by humans in the archaeological record. In general, paleobotanists study older plant material than archaeobotanists. Most paleobotanists study plants that are from the Pleistocene epoch (from about 2.6 million to 11,700 years ago) or older in age; in fact, evidence for land plants begins as far back as the Ordovician period, over 400 million years ago. Since archaeobotanists are specifically interested in the interactions between plants and people, they study material that dates maximally to the Pleistocene, but that is often younger. Modern humans (Homo sapiens) evolved about 200,000 years ago.
In order to study grasses in the past, paleontologists and archaeologists broadly rely on three types of evidence: macrofossils or macroremains (plant fragments that can be seen without the aid of a microscope, although a microscope may be used for detailed examination), microfossils or microremains (tiny plant fragments or structures that must be studied with a microscope), and isotopes (evidence from the chemical composition of organic remains like bones). They may also look at other types of evidence, like tools that indicate humans were cultivating and using grasses in the past.
Macroremains
In the paleontological record, grass macrofossil remains are relatively rare. Grass macrofossils include whole plants, rhizome or culm fragments, leaves, inflorescences, spikelets, grains, and bracts (i.e., glumes, lemmas). These are preserved as compressions and, especially beginning in the Miocene epoch (23 to 5.3 million years ago), as mummified or charcoalified remains that look similar to grass fragments found at some archaeological sites (see below). Grass macrofossils, including spikelets and leaf fragments, have also been found encased in amber (fossilized tree resin).
Plant macroremains at archaeological sites are likely to be charred (indicating contact with fire), and often include resistant reproductive material like seeds or fragments of seed coats, nutshells, cones, or other hard plant parts. Grass macroremains at archaeological sites are likely to include spikelets and grains.
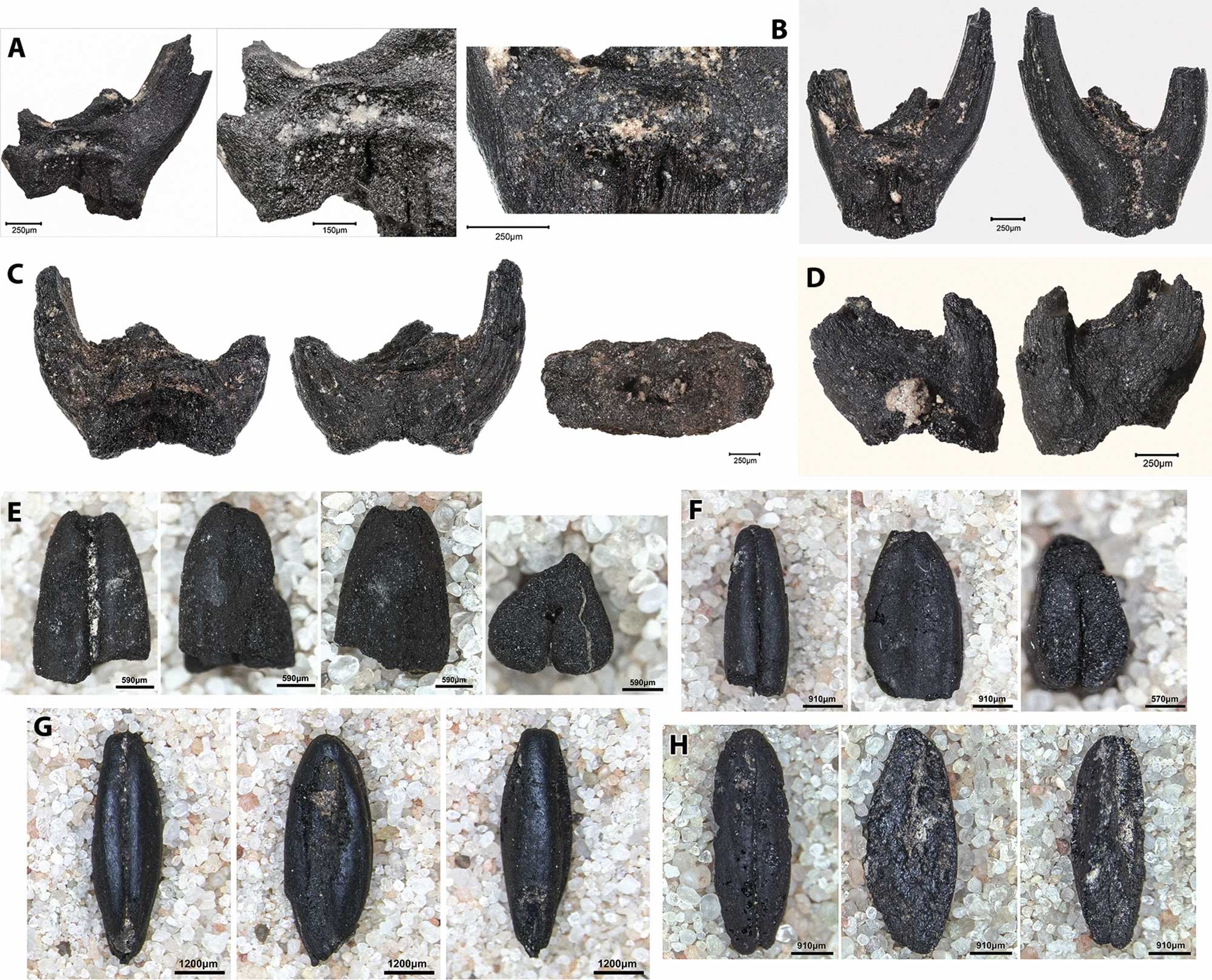
Archaeological remains of charred wheat (Triticum) from Gusir Höyük, Turkey, dating to about 10,500 to 10,300 years before present. A, B. Spikelet fork of wild einkorn wheat (T. boeoticum). C. Spikelet fork of wild emmer wheat (T. dicoccoides or T. turgidum subsp. dicoccoides). D. Wheat spikelet. E. Part of a wild emmer wheat grain. F-H. Grains of wild einkorn wheat. Source: Figure 7 in Kabukcu et al. (2021) Scientific Reports 11:2112 (Creative Commons Attribution 4.0 International license).
Pollen
Pollen is produced in the anthers of the grass flower. Pollen grains have a resistant outer wall made up of a hard substance called sporopollenin. The sporopollenin walls of pollen grains are often preserved in the paleontological and archaeological records. Scientists called palynologists break down sediments and treat them with acids in order to release the trapped pollen, which can be studied using a microscope.
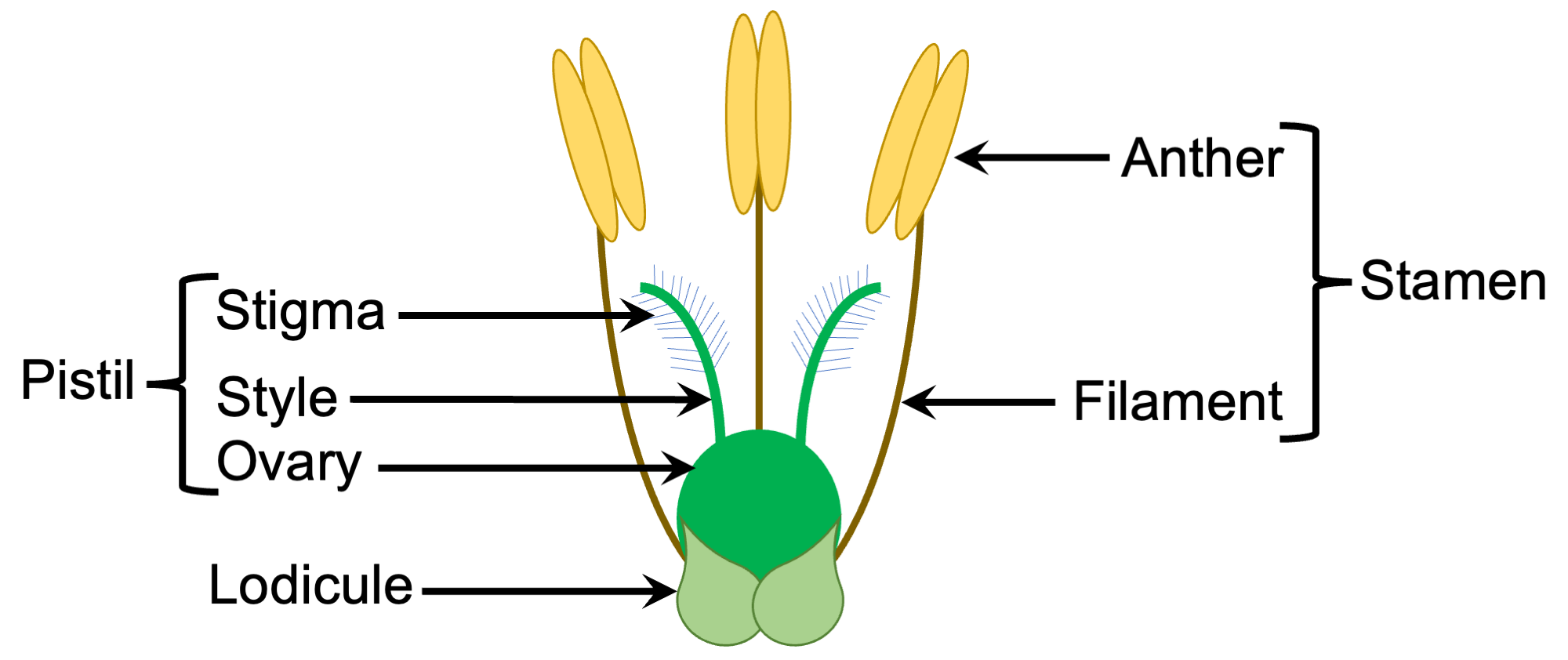
Diagram of a bisexual grass flower. Diagram by Elizabeth J. Hermsen (Evolution/Earth@Home).
Pollen morphology (form or appearance) is similar across members of the Grass Family (Poaceae). The typical grass pollen grain is spherical with a single pore surrounded by a raised rim; there are tiny bumps on its outer surface.
Unfortunately, because grass pollen is unvarying in its appearance, it is difficult to assign most dispersed grass pollen (pollen that has been released from anthers) to a genus or species of grass rather than simply to the Grass Family. Furthermore, pollen produced by some related plants looks similar to grass pollen, so the earliest occurrences of grass-like pollen in the fossil record must be verified using special techniques. Beginning in the Cenozoic (an era that spans 66 million years ago to present), pollen is a good indication that grass is present, but is often not a good indication of the type of grass.
Pollen is also difficult to identify beyond the family level in the archaeological record. The identification of domesticated grass pollen, especially pollen of maize (Zea mays), has been somewhat controversial and is considered most reliable when bolstered by other types of evidence (for example, starch and phytoliths). Notably, some grass grain crops (cereals) are self-pollinated (for example, wheat), meaning that they do not disperse their pollen by wind like many wild grasses; because of this, it is unlikely that pollen of these domesticated grasses will appear in the archaeological record.
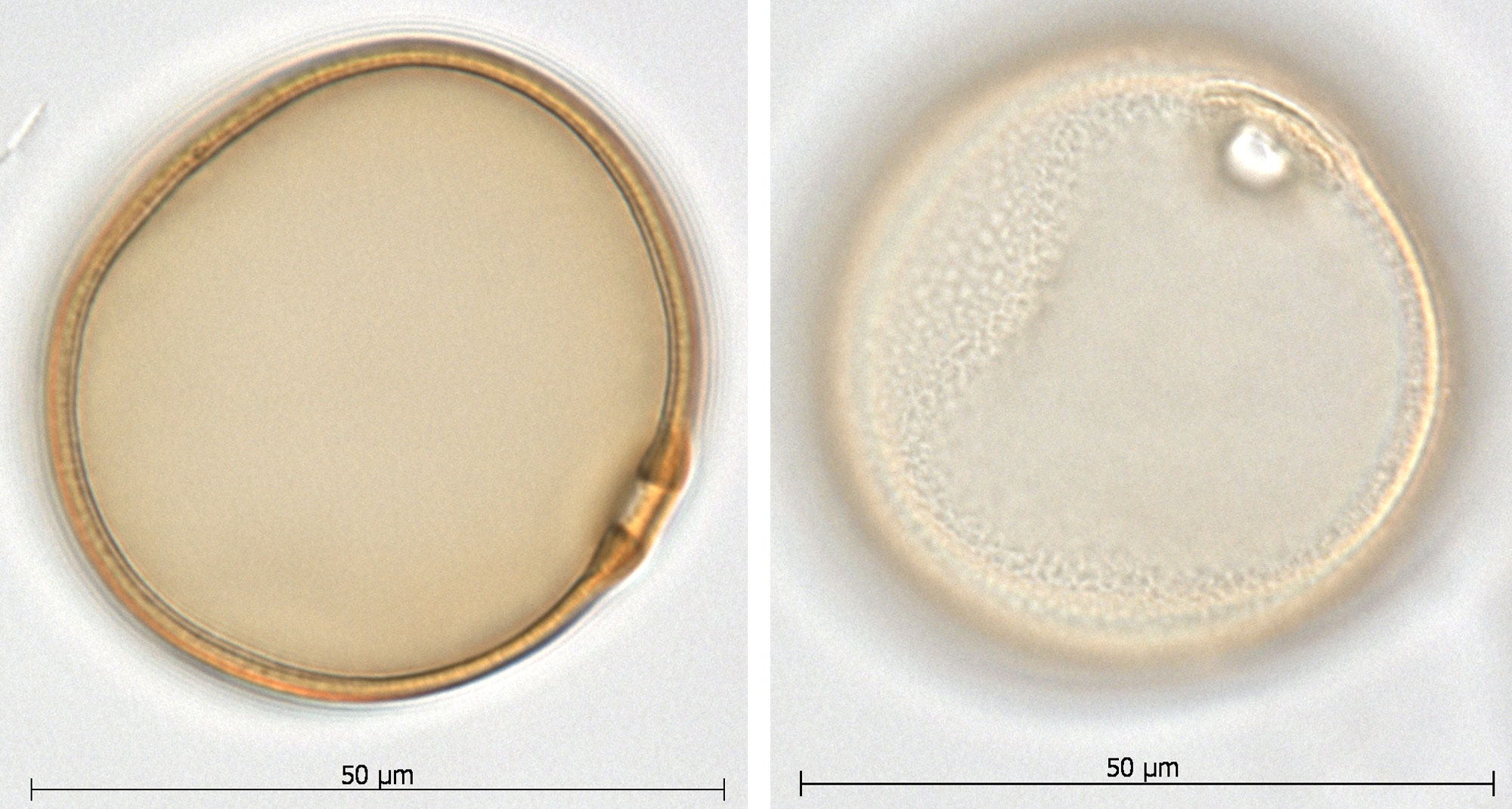
Modern pollen grain from tanglehead (Heteropogon contortus) as seen under a microscope. Credit: Images of ANU 17343 from the Australasian Pollen and Spore Atlas (Creative Commons Attribution-NonCommercial-ShareAlike 3.0 Unported license, images cropped and resized).
Epidermis and phytoliths
Phytoliths (a term meaning "plant stones") are tiny silica bodies (essentially, opal) that form in or between the cells of some plants. They occur in grasses and many other types of flowering plants, but are less common in non-flowering plant groups. Because each phytolith is about the size of a plant cell, phytoliths must be studied using a microscope.
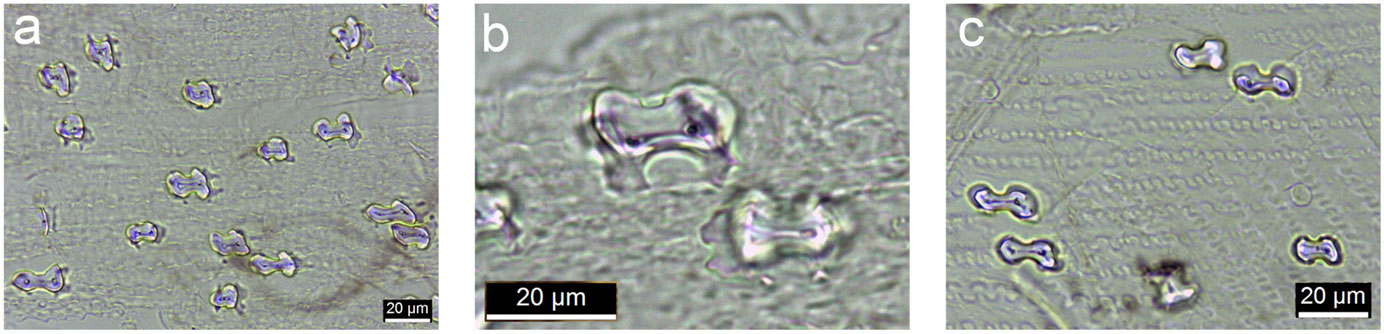
Bilobed silica bodies or phytoliths in the tissue of inflorescence bracts of a kangaroo grass (Themeda caudata). Source: Supplementary figure 6 from Ge et al. (2020) Frontiers in Plant Science (Creative Commons Attribution 4.0 International license, image cropped).
In grasses, many phytoliths come from the epidermis (the epidermis is the outer layer of cells that make up the "skin" covering the plant body) of the leaves and inflorescence bracts (in other words, the glumes, lemma, and palea, which are modified leaves). The cells that make up the epidermis are arranged in regular rows. They include elongated cells called long cells, shorter cells called short cells, and dumbbell-shaped guard cells and their subsidiary cells (flanking cells) that surround the stomata (pores for gas exchange). The epidermis may also have rows of bulliform cells; these specialized, large cells can fold the leaf up in dry conditions. Isolated grass epidermal fragments can be identified in the paleontological and archaeological records by the orientations and forms of the long and short cells, as well as the forms of the guard cells and subsidiary cells surrounding the stomata.
Silica bodies may be deposited in long cells and short cells. Short cells that contain silica bodies are called silica cells. Silica cells often contain silica bodies with distinctive shapes and may occur in conspicuous rows, often alternating with long cells. Suberin (a fatty material) may also be deposited in short cells called cork cells.
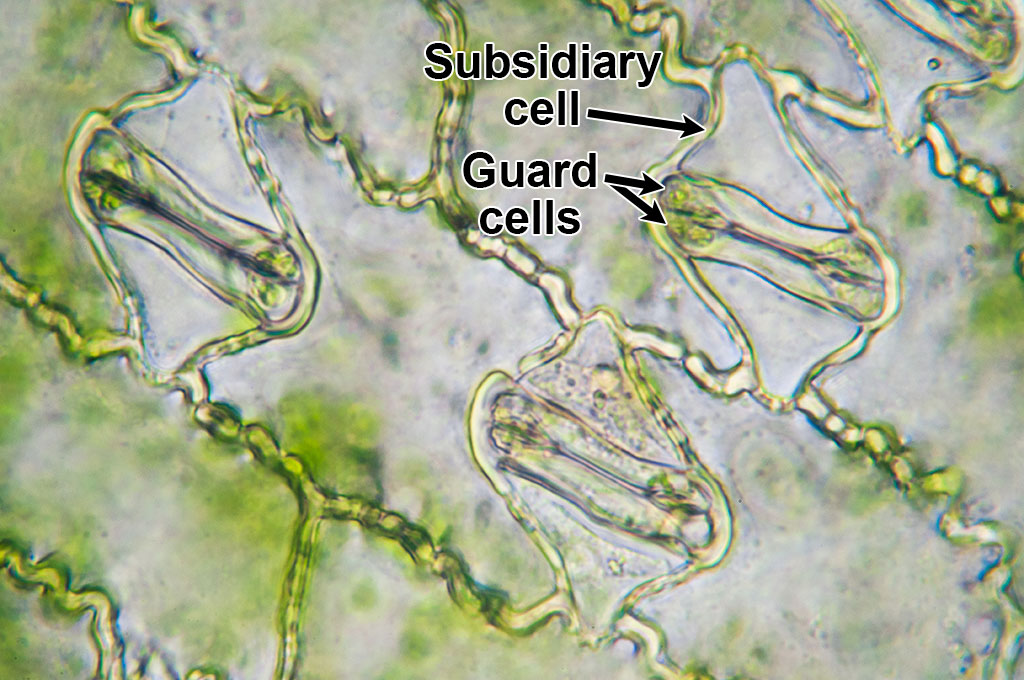
Microscopic image of maize (corn, Zea mays) epidermis showing three stomata with guard cells and subsidiary cells. In this image, the stomata are closed. Photo by Umberto Salvagnin (flickr, Creative Commons Attribution 2.0 Generic license, image labeled).
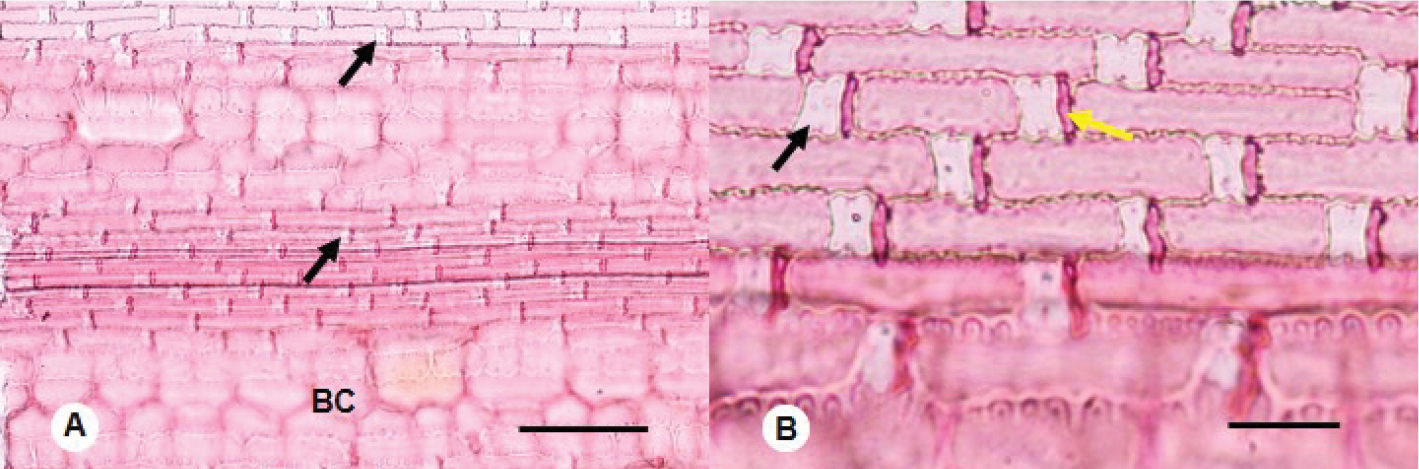
Epidermis of a herbaceous bamboo (Buergersiochloa bambusoides) at two magnifications under a microscope, treated with a stain. Left (A): Black arrows point to examples of silica cells. BC is a bulliform cell, a type of specialized cell involved in folding the leaf. Scale bar = 100 microns (0.1 millimeters). Right (B): Long cells with short cells between them. The black arrow indicates an example of a silica cell, the yellow arrow indicates an example of a cork cell (a short cell containing cork). The large, out-of-focus cells at the bottom of the image are bulliform cells. Scale bar = 25 microns (0.025 millimeters). Source: From figure 1 in Lima et al. (2021) Phytokeys 172: 135-143 (Creative Commons Attribution 4.0 International license, image cropped).
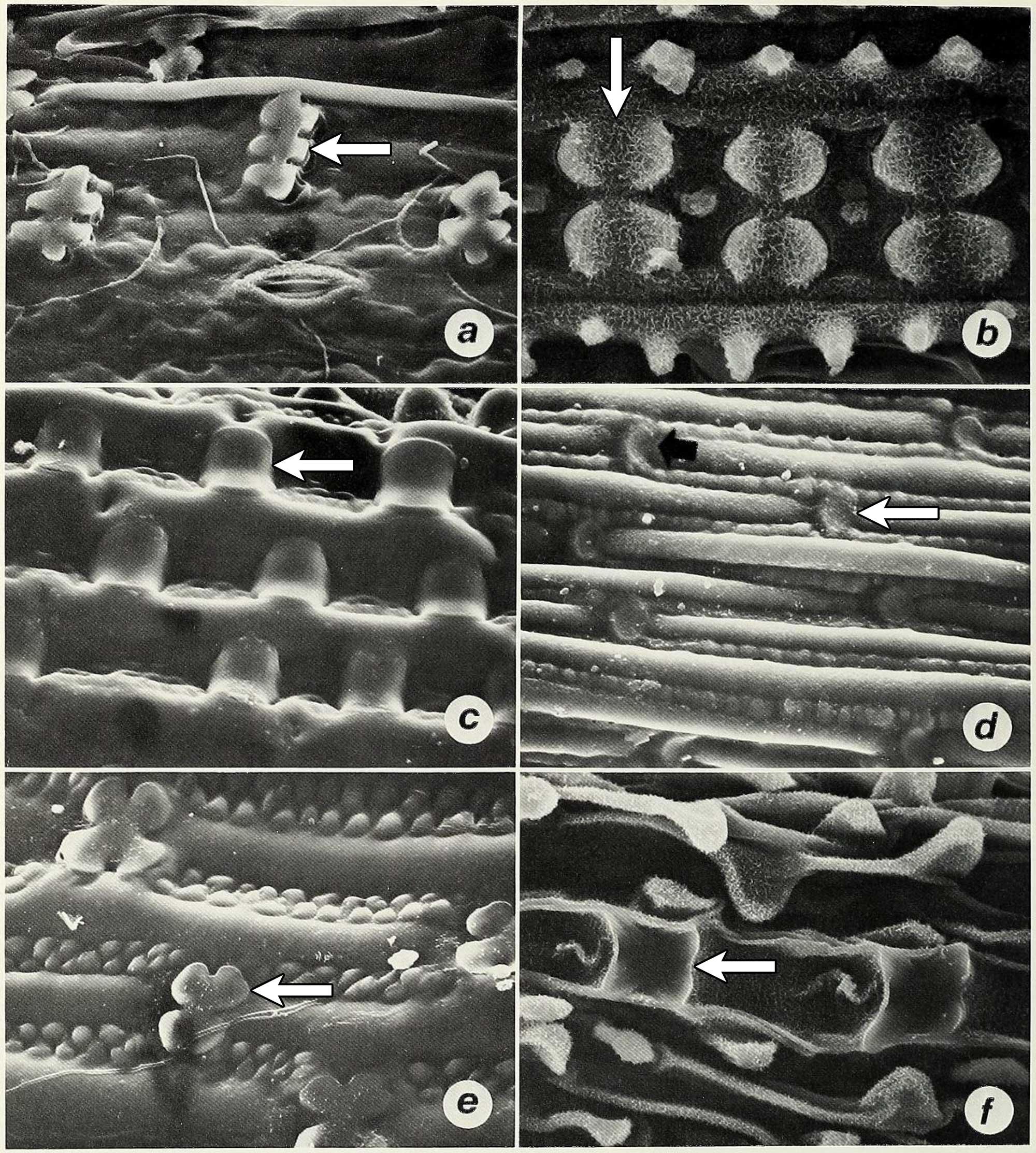
Scanning electron micrographs (magnified photographs taken with a scanning electron microscope) showing silica bodies in the epidermises of different grass species (examples of silica bodies indicated by arrows in each image). A. Polylobate in carycillo, a bamboo (Olyra latifolia). B. Bilobate or Oryza-type (rice-type) in African wild rice (Oryza barthii). C. Saddle in Chrysochloa hindsii. D. Rondel in Mediterranean needlegrass (Stipa dregeana). E. Cross in Phacelurus huillensis. F. Quadralobate Heteranthoecia guineensis. Source: Figure 5 from Palmer and Tucker (1981) Smithsonian Contributions to Botany 49 (Biodiversity Heritage Library, Creative Commons Attribution-NonCommercial-ShareAlike 4.0 International license, image cropped and white arrows added).
When a plant that contains silica bodies dies and its tissues decay, the silica bodies or phytoliths may persist to be deposited in soil or sediment. Phytoliths can be isolated from such soil or sediment using various techniques. Phytoliths may also be found in coprolites (fossil feces) or in archaeological artifacts, like containers and grinding stones.
Because silica can be deposited in both long and short cells, phytoliths come from both long cells and short cells. Phytoliths that come from short cells are sometimes called GSSC (grass silica short cell) phytoliths or GSSCPs. GSSCPs occur in various forms, such as acicular (pointed), bilobate (dumbbell-shaped), circular, cross-shaped, and saddle-shaped. The shapes of GSSCPs can be used to identify some major subgroups of grasses and sometimes can be used to identify grasses at even finer taxonomic levels, like genera.
Two editions of the International Code for Phytolith Nomenclature (ICPN) have now been published. This code seeks to standardize the terminology used to described phytoliths, which in the past has varied.
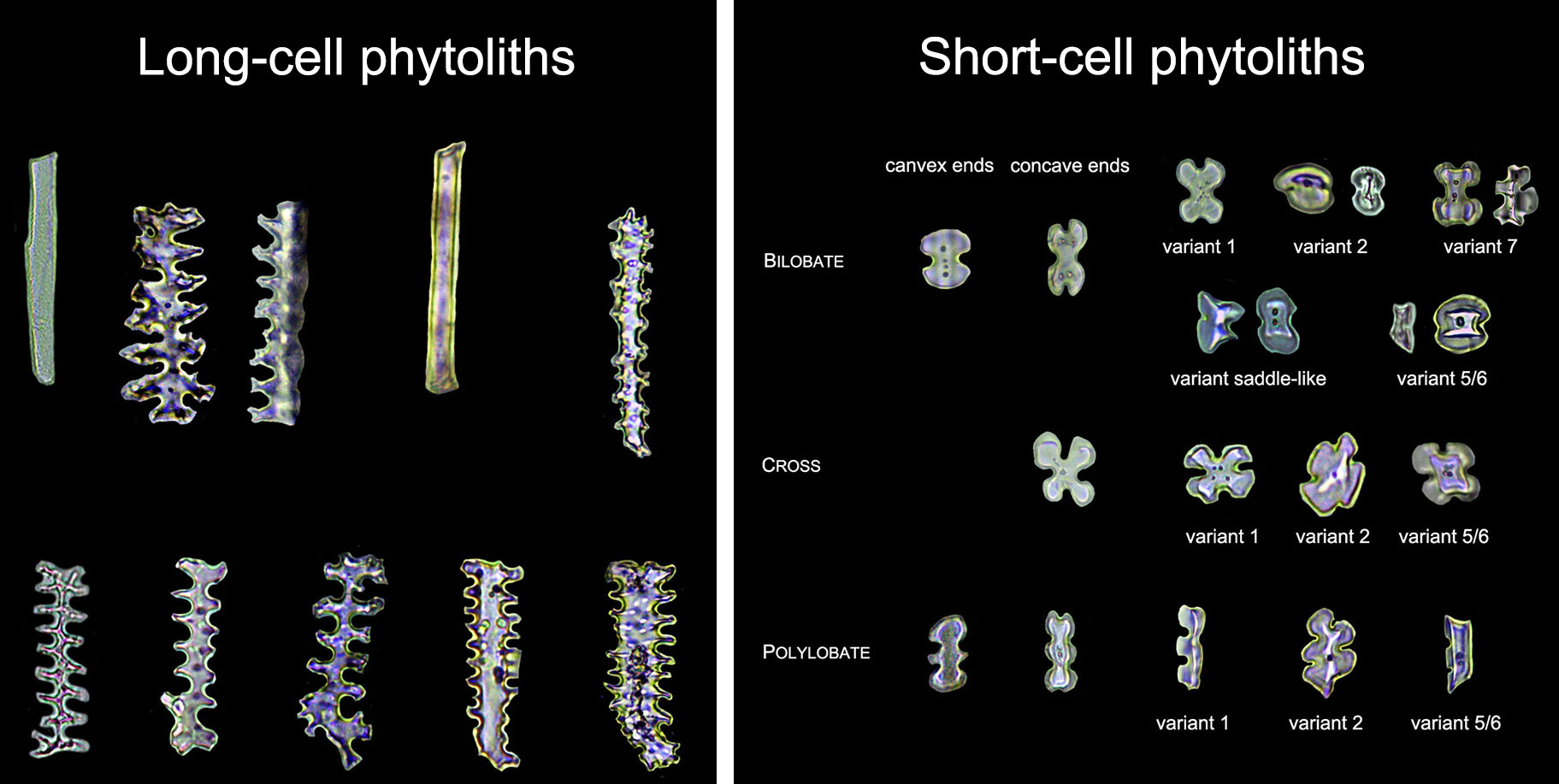
Examples of modern long-cell (left) and short-cell (right) phytoliths from the bracts of grasses in subfamily Panicoideae. Source: Figures 3 and 4 from Ge et al. (2020) Frontiers in Plant Science (Creative Commons Attribution 4.0 International license, images cropped and labels modified).
Starch grains
Starch is a type of complex carbohydrate that is found in many types of plants and serves as a major source of food for human beings. Plants store food as starch. In grasses, starch is stored primarily in the grain. In some other crops cultivated primarily for starch, it is stored elsewhere. For example, in potatoes (Solanum tuberosum), starch is stored is specialized underground stems called tubers; in cassava (Manihot esculenta), the starch is stored in fleshy roots.
Within plant cells, starch is stored in specialized, double-membrane-bound structures called plastids. Starch is made in amyloplasts and also found in chloroplasts, green plastids that are responsible for photosynthesis. Because starch grains are smaller than the size of a cell, they are very tiny and must be viewed using a microscope. Different types of lighting are used to reveal the different distinguishing features of starch grains.
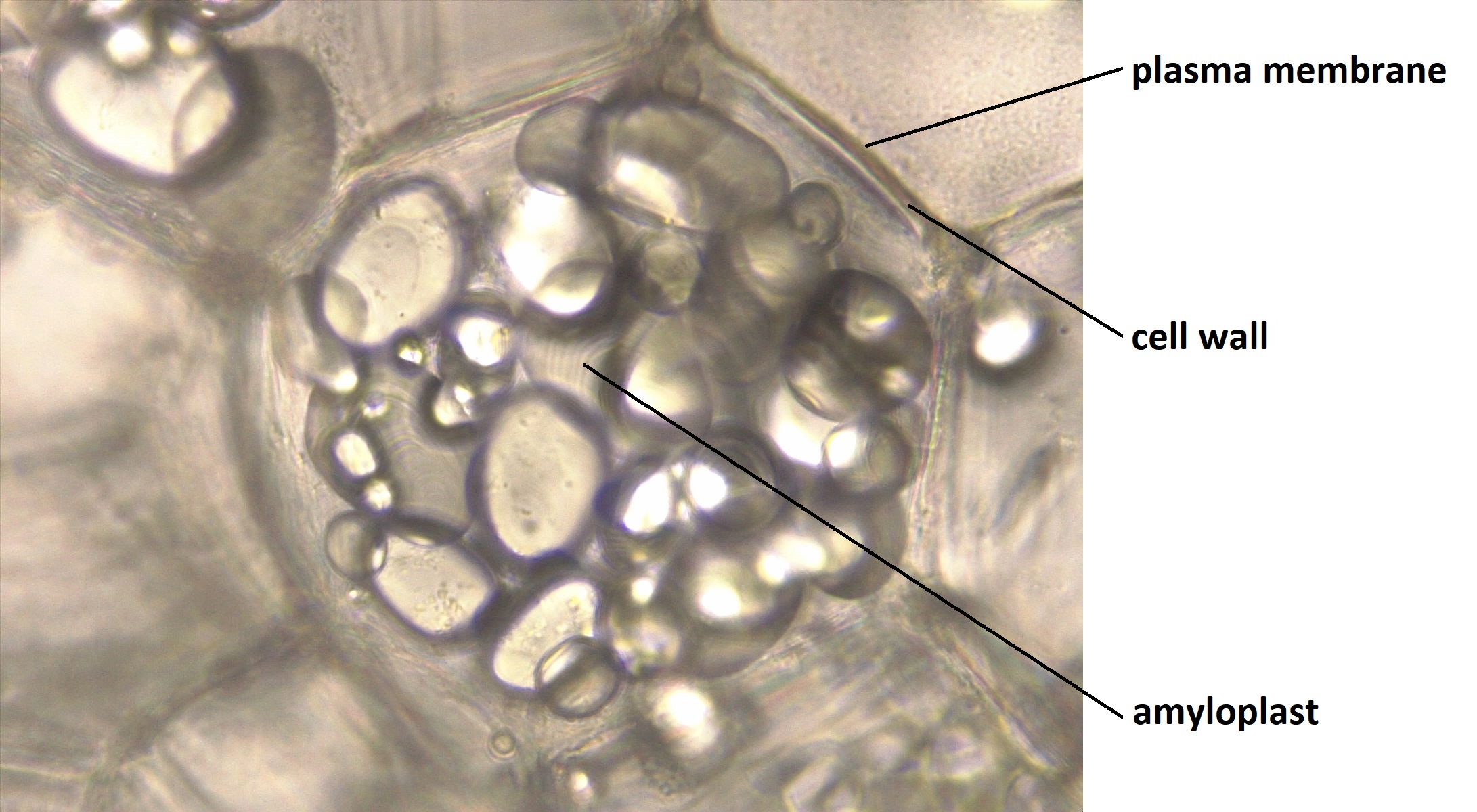
Amyloplasts containing starch in the cell of a potato. Photo by Melissa Ha (ASCCC OERI on flickr, Creative Commons Attribution-NonCommercial 2.0 Generic license).
Starch is resistant to decay and can be found in the archaeological record. Thus, starch grains are important sources of information about which plants people were eating and can even provide data about plant domestication. They can be found on tools used in food preparation and in food containers, and have even be recovered from human remains (like dental calculus, or tartar, on teeth).
Isolated starch grains can sometimes be identified to family level or below on the basis of their appearance. Some of the distinguishing features of starch grains are:
- Size, shape, and surface ornamentation.
- Hilum position. The hilum is the the origin point around which the starch in the grain is deposited. It may be centered or off-center.
- Shape of the central cavity, a depression around the hilum.
- The appearance of the extinction cross, a cross that can be seen only when a starch grain is viewed under cross-polarized light.
- Whether lamellae (concentric growth layers) or fissures (radiating cracks) can be seen.
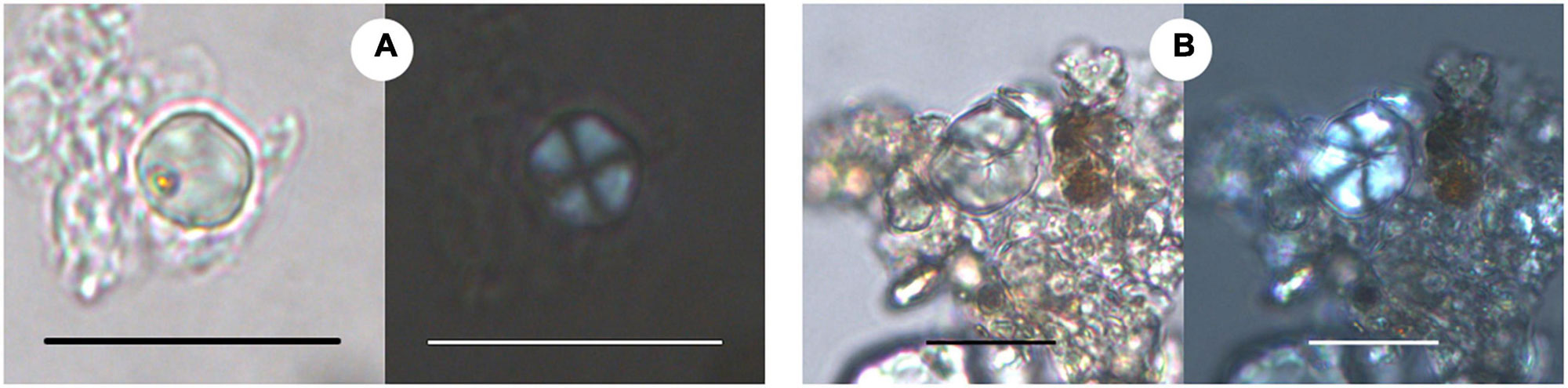
Ancient starch grains recovered from pottery found at archaeological sites in Gujarat, India, dating between about 3300 and 2000 years B.C. Each pair of images shows the grains under brightfield illumination (regular transmitted light) and cross-polarized light (showing the extinction cross). Left panels (A): Starch from millet (Millet Tribe/Tribe Paniceae). Right panels (B): Starch from Job's tears (Coix lachyrma-jobi). Scale bars are 20 microns (0.02 millimeters). Source: Images from figure 3 in García-Granero et al. (2022) Frontiers in Ecology and Evolution 10:840199 (Creative Commons Attribution 4.0 International license, image cropped).
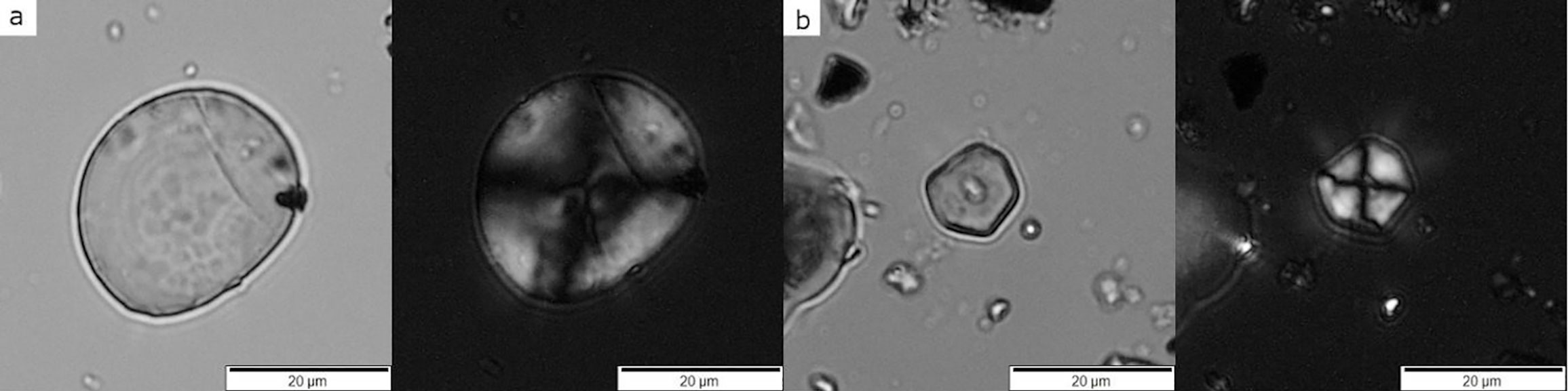
Ancient starch grains recovered from grinding tools at the Neolithic archaeological site Çatalhöyük in Turkey, dating between about 6700 and 6300 years B.C. Each pair of images shows the grains under brightfield illumination (regular transmitted light) and cross-polarized light (showing the extinction cross). Left panels (A): Starch from a member of the Wheatgrass Tribe (Tribe Triticeae). Right panels (B): Starch from a member of the Millet Tribe (Tribe Paniceae). Source: Images from figure 4 in Santiago-Marrero et al. (2021) PLoS ONE 16(6):E0252312 (Creative Commons Attribution 4.0 International license, image cropped).
Indirect evidence
Indirect evidence for grasses includes evidence that suggests that grasses are present, even if the physical structures of the grass are not preserved. Evidence from animal tooth shape and evidence from isotopes are types of indirect evidence discussed below. Human tools used for processing grasses for food would be another type of indirect evidence for grasses in the archaeological record.
Mammal tooth morphology (shape or form)
Grazing mammals are mammals like cows and horses that feed on plants that grow near the ground, like grasses. They tend to have hypsodont, or high-crowned, teeth. Scientists once thought high-crowned teeth evolved in grazing mammals to allow them to eat grass tissues that contain abrasive silica bodies (phytoliths). High-crowned teeth are found in animals that consume abrasive material because these long teeth take more time to wear down than low-crowned teeth.
Newer research shows, however, that the first evolution of high-crowned teeth came before the widespread expansion of grasslands during the Miocene epoch (spanning about 23 to 5.3 million years ago). Instead, high-crowned teeth are correlated with the presence of grit in the diet of plant-eating mammals. Grit may include, for example, particles of dirt. High-crowned teeth are now thought to be correlated with dry and open environments in which mammals ingest a lot of grit as they eat plants. Nevertheless, beginning in the Miocene, such open biomes were increasingly widespread and grass-dominated.
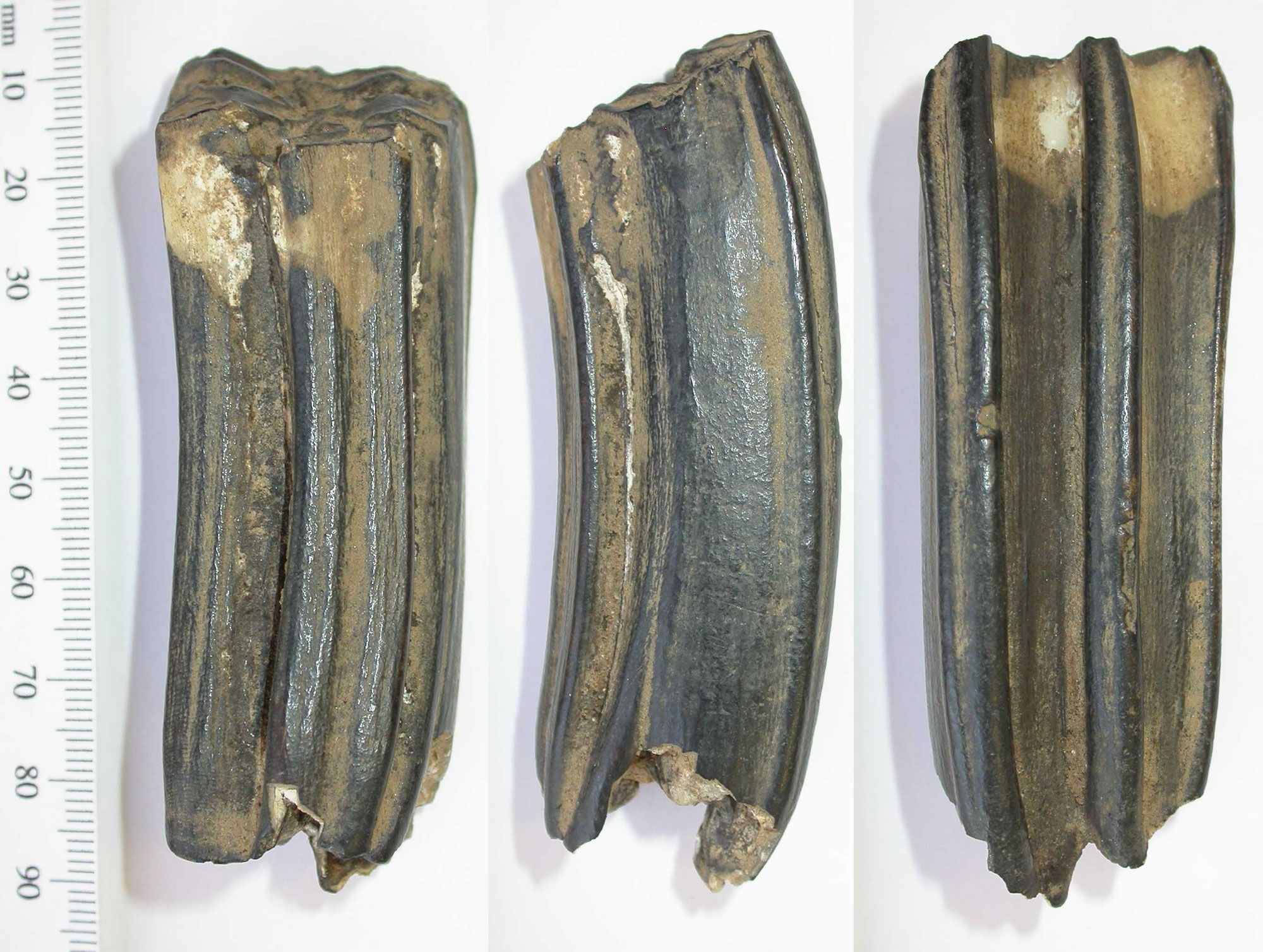
Three views of a high-crowned horse tooth from the Paleolithic (late Pliocene to Pleistocene), Derbyshire County, England. Photo by Rachel Atherton, Derby Museums Trust (Wikimedia Commons, Creative Commons Attribution-ShareAlike 2.0 Generic license, image resized).
Carbon isotopes
Through photosynthesis, plants convert carbon dioxide (CO2) and water into food (a carbohydrate) in the presence of sunlight (energy). The carbon in the carbohydrate may eventually be incorporated into plant tissues. If a plant is consumed by an animal, the carbon from the plant will be incorporated into the animal's tissues. If that animal is consumed by another animal, the carbon will then be incoporated into that animal's tissues, and so on.
Carbon is an element. An element is a type of atom defined by the number of protons, or positively charged particles, in its nucleus. A hydrogen atom, for example, has one proton, a carbon atom has six, and an oxygen atom has eight. In addition to protons, atoms have neutrons, or neutral particles, in their nuclei. Typically, the number of protons and the number of neutrons in the nucleus of an atom is equal.
One way to study grasses is using indirect evidence from stable isotopes. Isotopes are forms of an element with different numbers of neutrons in the nucleus. For example, a typical carbon atom has six neutrons, but some carbon isotopes have seven or eight. Thus, most carbon atoms are carbon-12 (12C) and have 12 particles, six protons plus six neutrons, in their nuclei. 13C has 13 particles, six protons and seven neutrons; 14C has 14 particles, six protons and eight neutrons. Note that in all cases the number of protons defines the identity of the element, which is carbon.
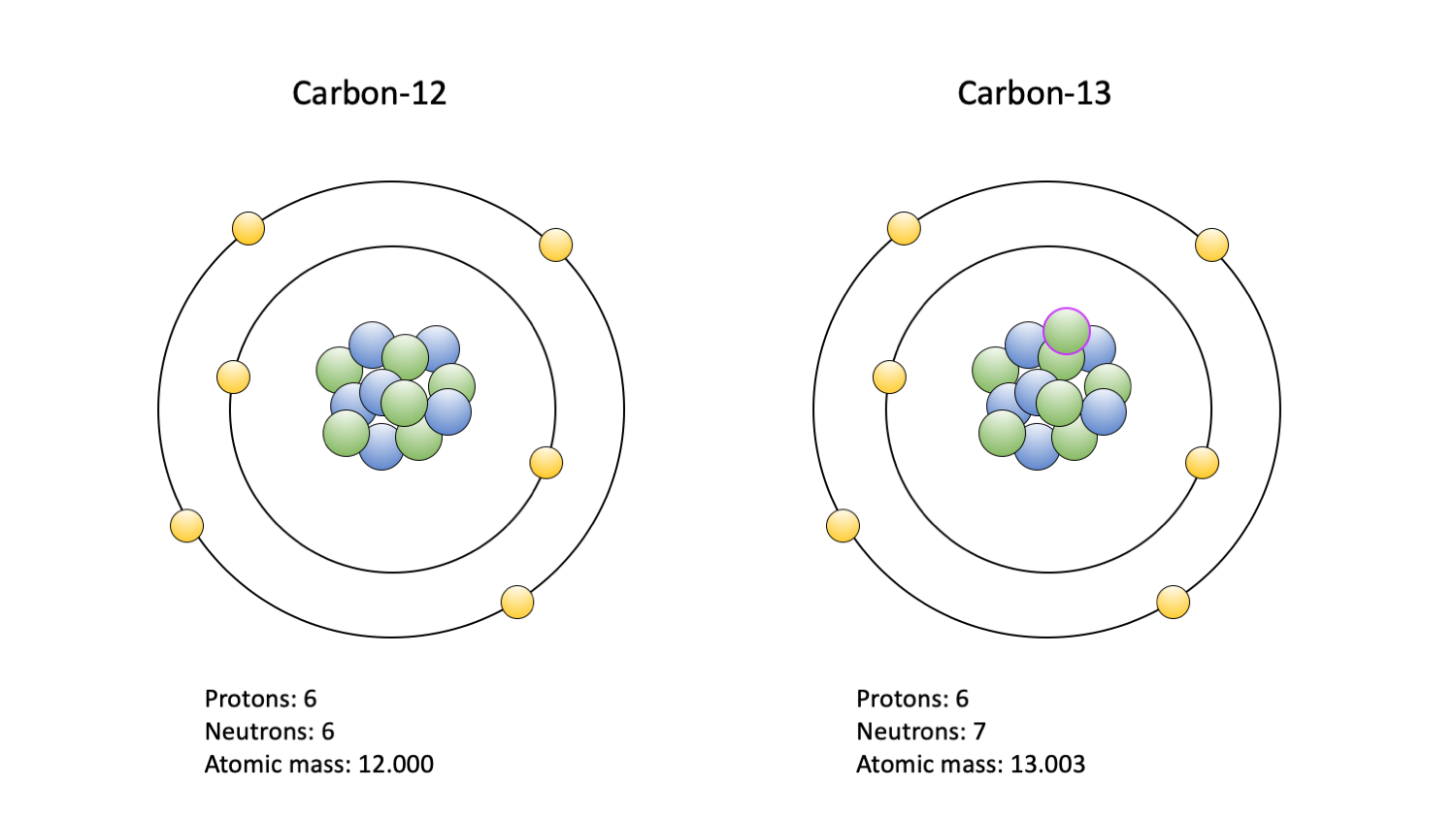
"The two carbon isotopes commonly used in paleoecological studies: 12C (left) and 13C (right). Carbon-14 is also used, but primarly to date fossils; it is not depicted. Protons are blue; neutrons are green, with a purple outline indicating an extra in the 13C isotope nucleus; electrons are yellow." Figure and caption by Jansen Smith (Digital Atlas of Ancient Life, Creative Commons Attribution-NonCommercial-ShareAlike 4.0 International License).
Stable isotopes exist, as the name suggests, in a stable form. Unstable radioactive isotopes do not exist in a stable form and undergo radioactive decay. 12C and 13C are stable; 14C is unstable. When 14C decays, it becomes nitrogen-14 (14N); nitrogen has seven protons in its nucleus.
Grasses have two types of photosynthesis. Most grasses are C3, whereas a large minority (about 4500 species) are C4. C3 grasses have the typical photosynthetic pathway found in most plants; C4 grasses have a different, more specialized pathway. C4 plants are able to use more CO2 molecules with 13C isotopes that C3 plants, which use a greater proportion of CO2 molecules with 12C isotopes. The ratio of 13C to 12C thus differs in C3 and C4 plants, and can be used to detect the presence of C4 plants in the fossil record.
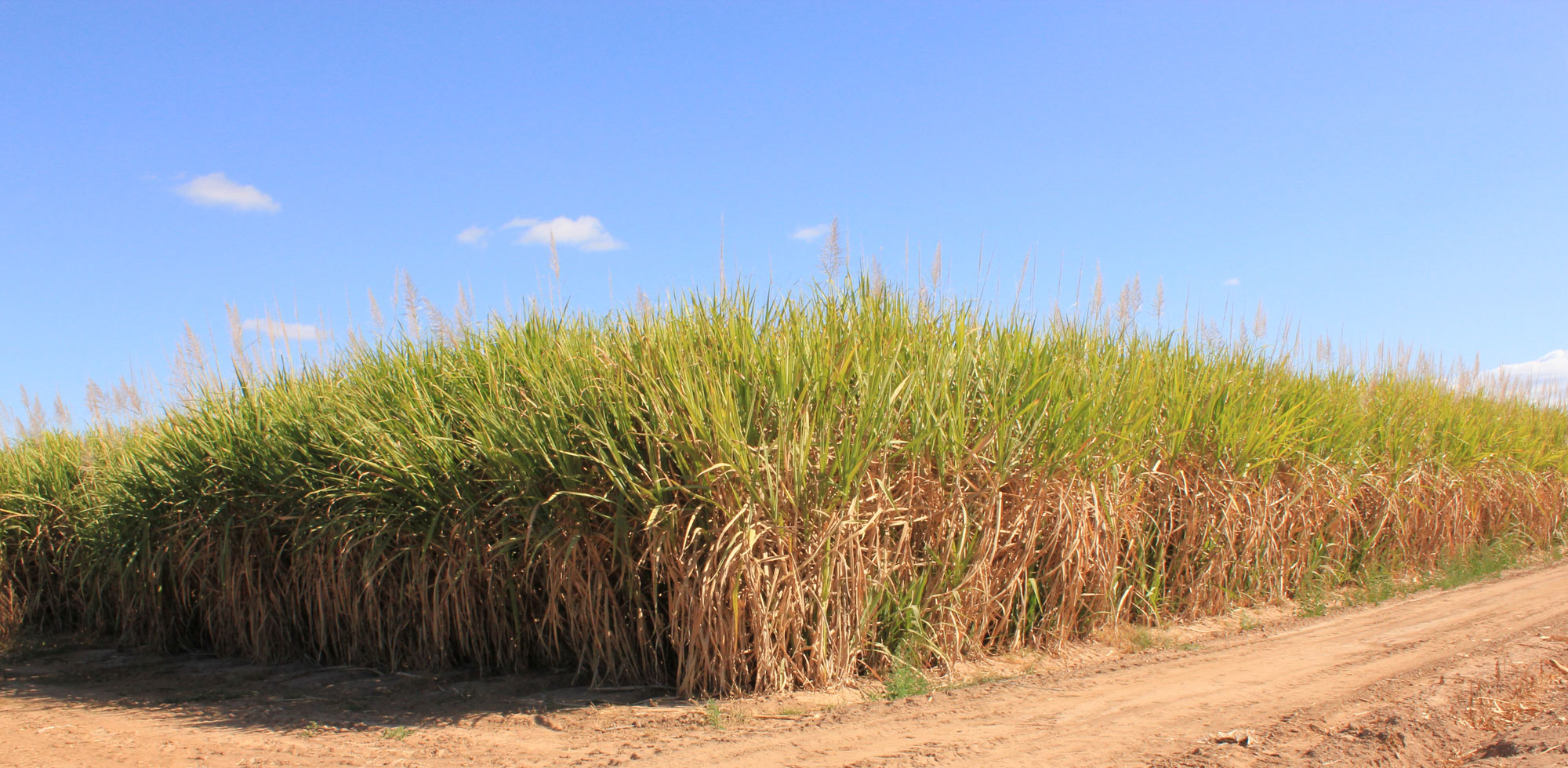
Field of sugar cane (Saccharum officianarum), Queensland, Australia. Photo by Richard Riley (flickr, Creative Commons Attribution 2.0 Generic license, image cropped and resized).
13C-12C ratios remain constant once they are incorporated into organic structures, like plant or animal tissues. 13C-12C ratios are often studied in the tissues (often teeth) of animals in the paleontological and archaeological records in order to understand their diets. Animals' diets reflect the plants growing in the environments they inhabited, and, thus, their diets also inform our understanding of paleoenvironments. In the case of humans living in societies that depended on agriculture, 13C-12C ratios reflect the types of plants that they cultivated for food. 13C-12C ratios can also be studied using carbon in paleosols (ancient soils), where they reflect the carbon ratios of the plants that were growing in the soil.
C4 photosynthesis occurs in a small number of plant groups, and grasses make up the group with the most C4 plants. Because of this, researchers examining stable carbon isotopes often assume that carbon ratios indicating C4 photosynthesis reflect the presence or consumption of C4 grasses.
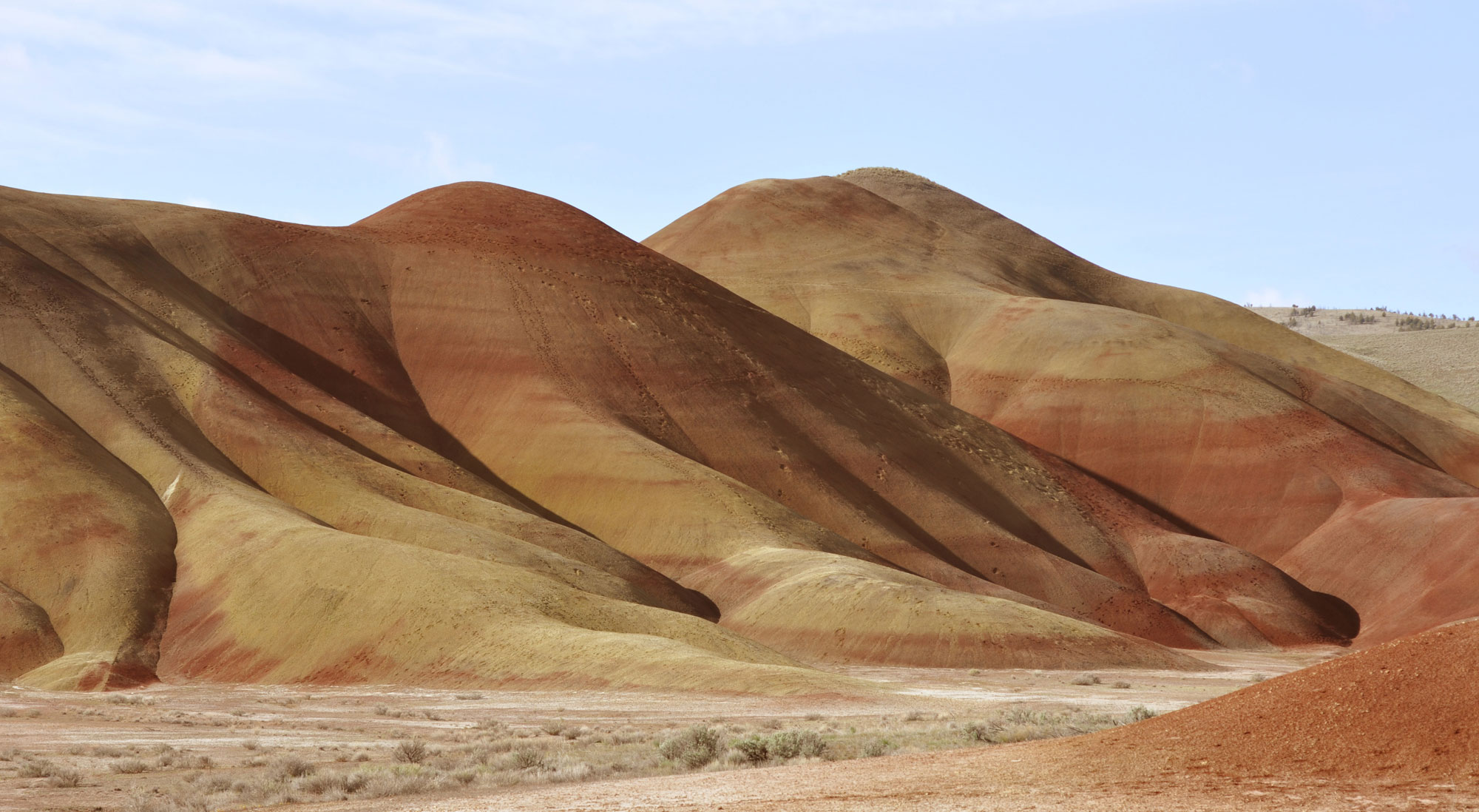
Cenozoic paleosols in the Painted Hills, Oregon, U.S.A. The red bands are woodland paleosols. Photo by Retallack (Wikimedia Commons, Creative Commons Attribution-ShareAlike 3.0 Unported license, image cropped and resized).
Resources
Websites
Additional resources from the Paleontological Research Institution
Digital Atlas of Ancient Life: Biogeochemical Analysis and Paleoecology (by Jansen Smith)
Other websites
Behind the Science: C3 or C4, which one are you? (H. Gregory, The Leakey Foundation): https://leakeyfoundation.org/2015behind-the-science-c3-or-c4-which-one-are-you/
The technical details: Radioactive decay (NOAA Global Monitoring Laboratory): https://gml.noaa.gov/ccgg/isotopes/decay.html
Phytolith nomenclature
ICPN Working Group [Madella, M., A. Alexandre, and T. Ball]. 2005. International Code for Phytolith Nomenclature 1.0. Annals of Botany 96: 253-260. https://doi.org/10.1093/aob/mci172
International Committee for Phytolith Taxonomy [K. Neumann, C. A. E. Strömberg, T. Ball, R. M. Albert, L. Vrydaghs, and L. S. Cummings]. 2019. International Code for Phytolith Nomenclature (ICPN) 2.0. Annals of Botany 124: 189-199. https://doi.org/10.1093/aob/mcz064
Scientific articles and books
Evert, R. F., and S. E. Eichhorn. 2013. Raven Biology of Plants, 8th ed. W.H. Freeman and Company Publishers, New York, New York.
Ge, Y., H. Lu, J. Zhang, C. Wang, and X. Gao. 2020. Phytoliths in inflorescence bracts: preliminary results of an investigation on common Panicoideae plants in China. Frontiers in Plant Science 10:1736. https://doi.org/10.3389/fpls.2019.01736
Jardine, P. E., C. M. Janis, S. Shaney, and M. J. Benton. 2012. Grit not grass: Concordant patterns of early origin of hypsodonty in Great Plains ungulates and Glires. Palaeogeography, Palaeoclimatology, and Palaeoecology 365-366: 1-10. https://doi.org/10.1016/j.palaeo.2012.09.001
Kellogg, E. A. 2015. Flowering Plants, Monocots, Poaceae. The Families and Genera of Vascular Plants 13, 416 pp. (K. Kubitzki, ed.). Springer International Publishing, Switzerland. https://doi.org/10.1007/978-3-319-15332-2_1
Louderback, L. A., S. Wilks, N. M. Herzog, G. H. Brown, K. Joyce, and B. M. Pavlik. 2022. Morphometric identification of starch granules from archaeological contexts: Diagnostic characteristics of seven major North American plant families. Frontiers in Earth Science 10:89183. https://doi.org/10.3389/feart.2022.897183
Miller, N. 1995. Archaeobotany: Macroremains. American Journal of Archaeology 99: 91-93. https://doi.org/10.2307/506880
Palmer, P. G., and A. E. Tucker. 1981. A scanning electron microscope survey of the epidermis of East African Grasses, I. Smithsonian Contributions to Botany 49, 84 pp.
Pipnero, D. R. 1988. Phytolith analysis: An archaeological and geological perspective. Academic Press, Inc., San Diego, California.
Rudall, P. J., C. J. Prychid, and T. Gregory. 2014. Epidermal patterning and silica phytoliths in grasses: An evolutionary history. Botanical Review 80: 59-71. https://doi.org/10.1007/s12229-014-9133-3
Semprebon, G. M., F. Rivals, and C. M. Janis. 2019. The role of grass vs. exogenous abrasive in the paleodieteary patterns of North American ungulates. Frontiers in Ecology and Evolution 7:65. https://doi.org/10.3389/fevo.2019.00065
Strömberg, C. A. E. 2011. Evolution of grasses and grassland ecosystems. Annual Review of Earth and Planetary Sciences 39: 517-544. https://doi.org/10.1146/annurev-earth-040809-152402
Strömberg, C. A. E., R. E. Dunn, C. Crifò, and E. B. Harris. 2018. Phytoliths in paleoecology: Analytical considerations, current use, and future directions. Pp. 235-287 in D. A. Croft et al., eds., Methods in Paleoecology: Reconstructing Cenozic terrestrial ecosystems and ecological communities. https://doi.org/10.1007/978-3-319-94265-0_12
Twiss, P. C., E. Suess, and R. M. Smith. 1969. Morphological classification of grass phytoliths. Proceedings of the Soil Science Society of America 33: 109-115.