Page snapshot: Introduction to the genetics of grasses, including DNA, genes, chromosomes, inheritance, and polyploidy.
Topics covered on this page: Introduction; DNA; Sexual reproduction and variation; Meiosis; Meiosis I; Meiosis II; Fertilization; Laws of Inheritance; Law of Segregation; Law of Independent Assortment; Law of Dominance; Other sources of variation; Mutations; Transposition; Polyploidy; Resources.
Credits: Funded by the National Science Foundation. Any opinions, findings, and conclusions or recommendations expressed in this material are those of the author(s) and do not necessarily reflect the views of the National Science Foundation. This page incorporates content prepared for a revised manuscript of the Teacher-Friendly Guide to the Evolution Maize (Carlyn Buckler, Dhyan Palanichamy, and Andrielle Swaby, 2019). Additional content and revisions by Elizabeth J. Hermsen (2022-2023).
Updates: Page last updated January 19, 2023.
Image above: Diagrams illustrating the make-up of a chromosome. Illustration courtesy of National Human Genome Research Institute (public domain).
Introduction
In the same way that we inherit traits from our parents, genes are passed from one generation to another in plants and other organisms. Genes are the units of heredity; they are the templates for proteins that make up an organism. Genes occur on chromosomes. Chromosomes are strands of deoxyribonucleic acid, or DNA, that encode the genes.
The term genotype refers to the entire genetic makeup of an organism, while the term phenotype refers to an organism’s physical appearance. The phenotype of an organism is the result, or expression, of the genotype that we can see or measure. For example, blue eyes versus brown eyes in humans, or yellow kernels versus red kernels in maize.
How is diversity in phenotype created? During the process of inheritance, segments of DNA are shuffled and recombined to form new variations, such that offspring are genetically and phenotypically different than their parents. Thus, each individual that results from sexual reproduction has a unique combination of traits distinct from those of its parents.
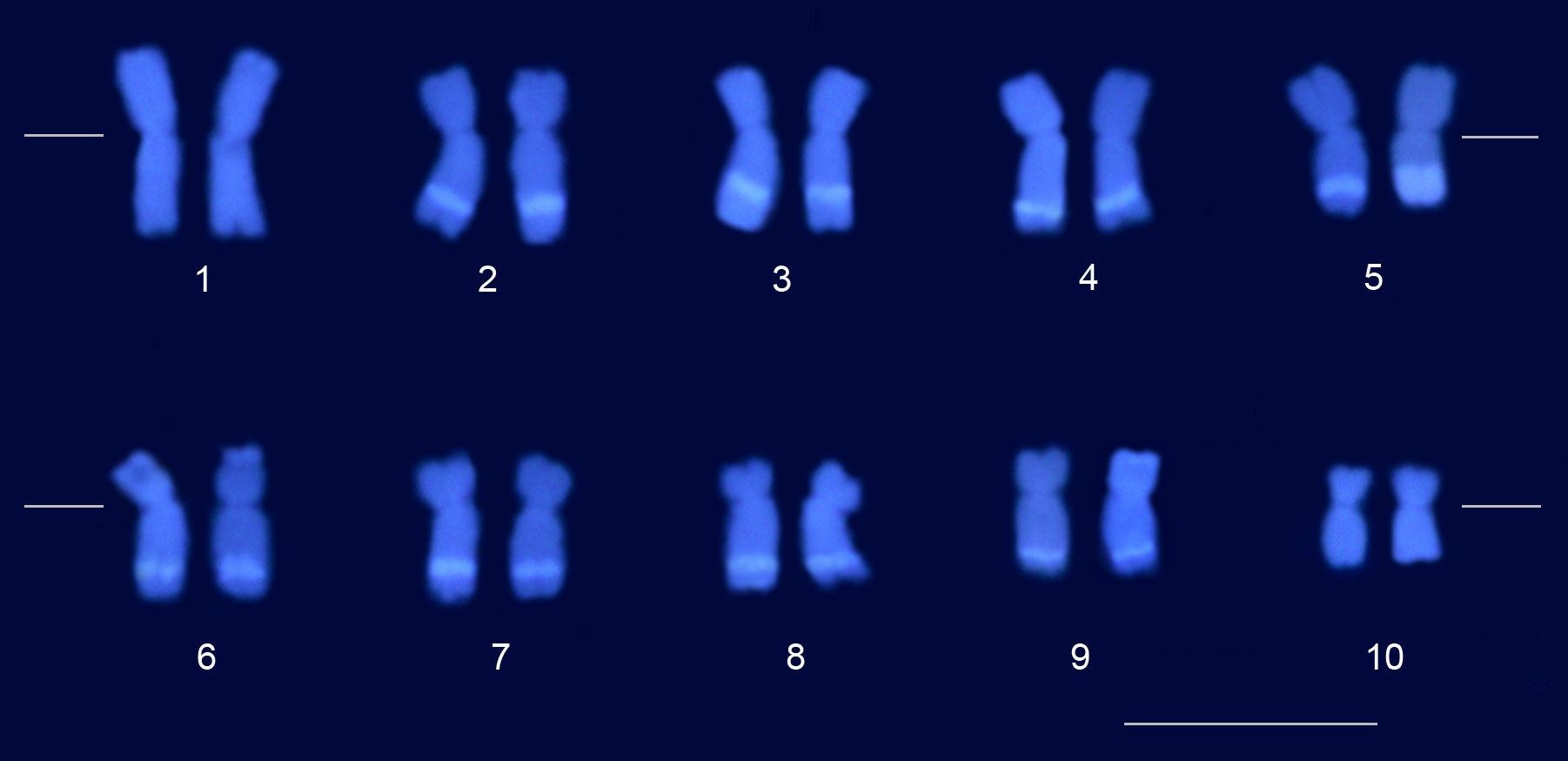
Maize (Zea mays 'AL Bandeirante') chromosomes. Each maize plant has two copies of each of 10 chromosomes, for a total of 20 chromosomes. Scale bar (horizontal line, lower right) = 0.01 mm. Source: Figure S1 from Silva et al. (2018) PLoS ONE 13(1): e0190428 (Creative Commons Attribution 4.0 International license, image cropped).
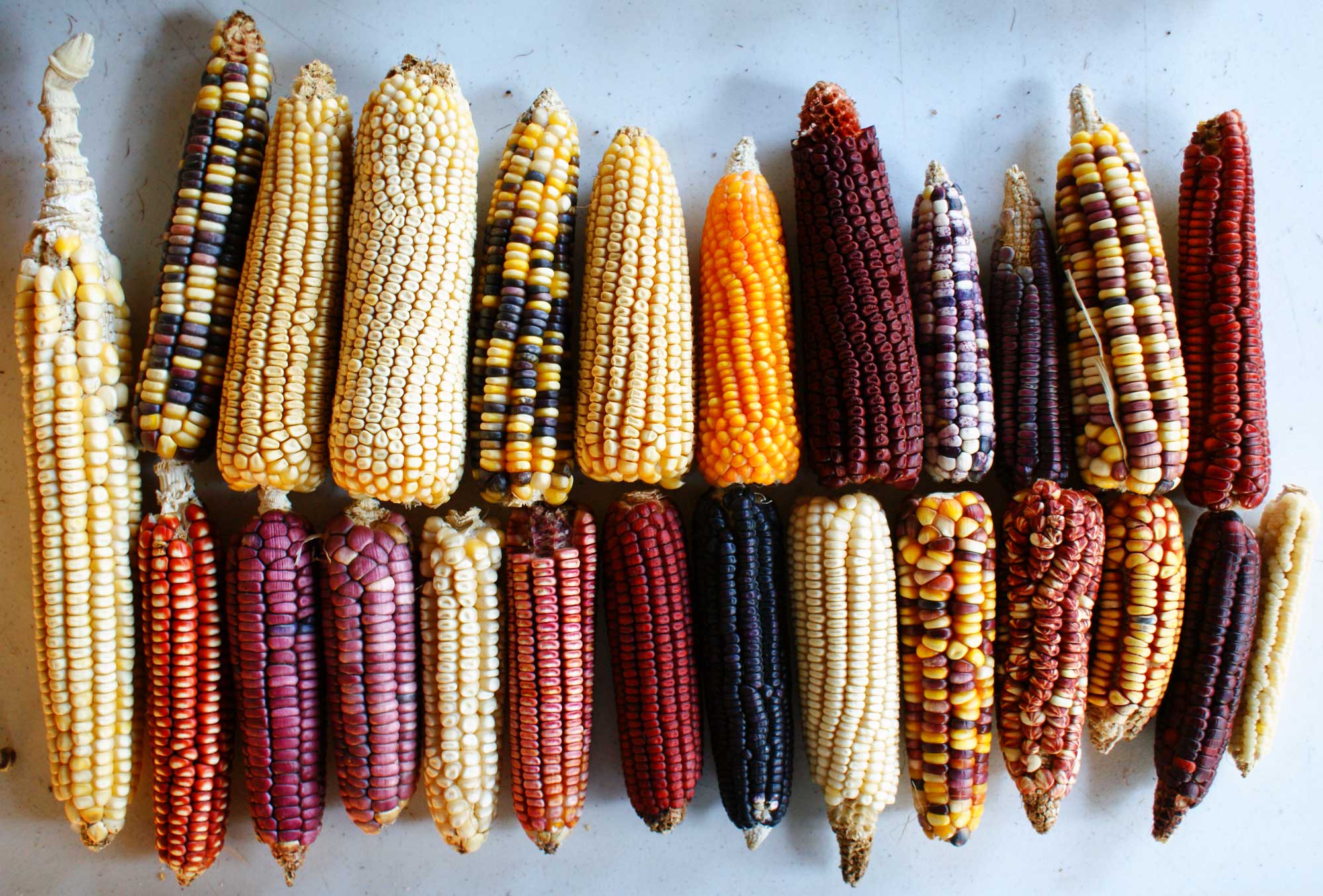
Ears of different corn varieties from Mexico showing variations in phenotypes, most noticeable in kernel pigmentation. Photo by Feria de Productores on flickr (Creative Commons Attribution 2.0 Generic license, image cropped and resized).
DNA
DNA is a two-stranded, string-like molecule composed of a backbone of pentose sugars (5-carbon sugars) and phosphate groups, with nucleotide bases (also called simply nucleotides or bases) attached. There are four possible nucleotides: adenine, thymine, guanine and cytosine, usually known simply as A, T, G, and C. The shapes of the nucleotides are complementary; A and T bind only to each other, and G and C bind only to each other. Thus, if we know the sequence of one of the strands of DNA, we can deduce the sequence of the other strand. For example, if we know that one strand has a sequence of ACCGT, then we deduce that the complementary strand has an opposing sequence of TGGCA. This complementary structure also makes it possible for DNA to replicate itself.
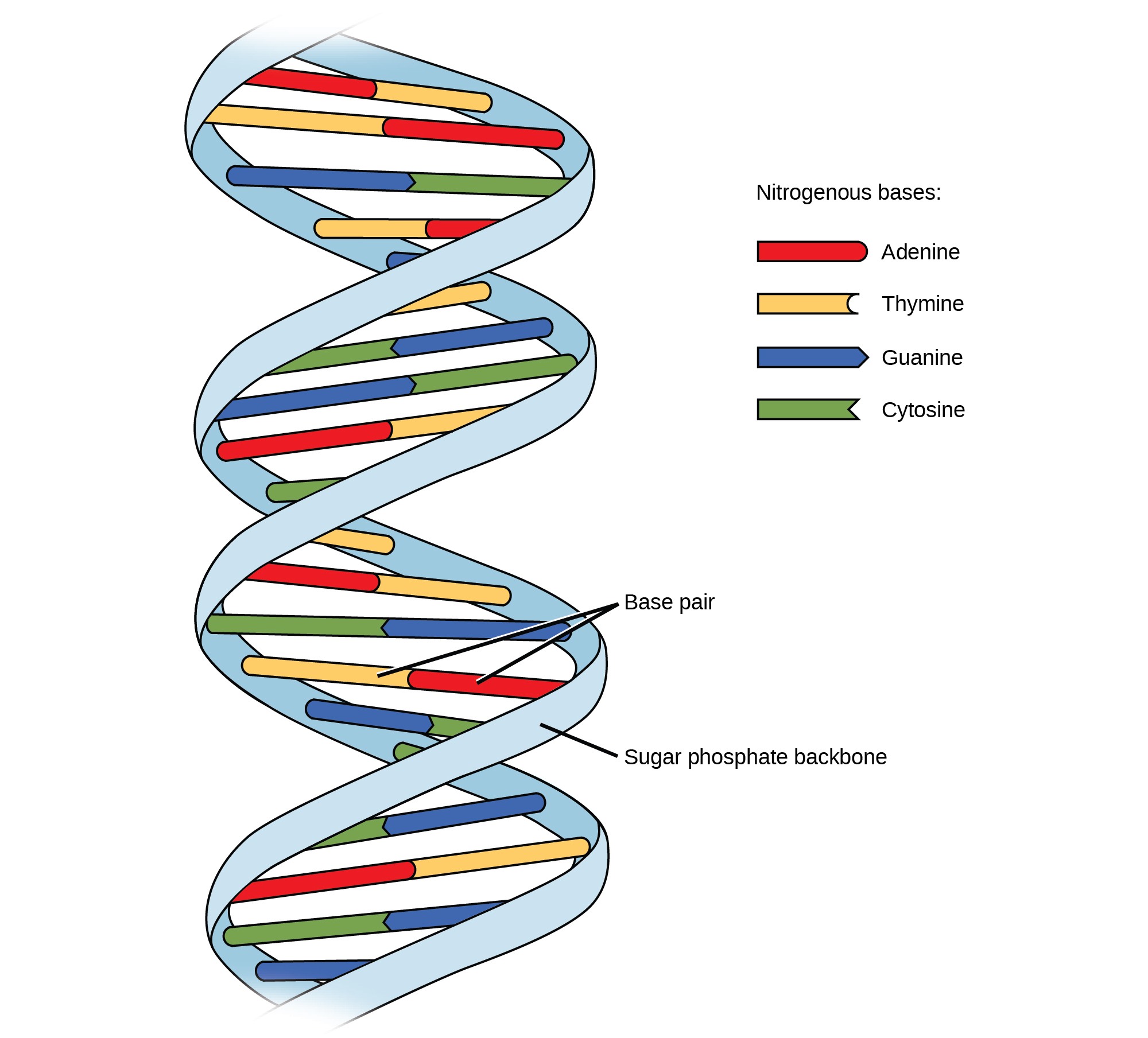
Illustration of a strand of DNA showing the structure of the double helix and the pairing of bases in the molecule. Illustration by OpenStaxCollege (Wikimedia Commons, Creative Commons Attribution 3.0 Unported license).
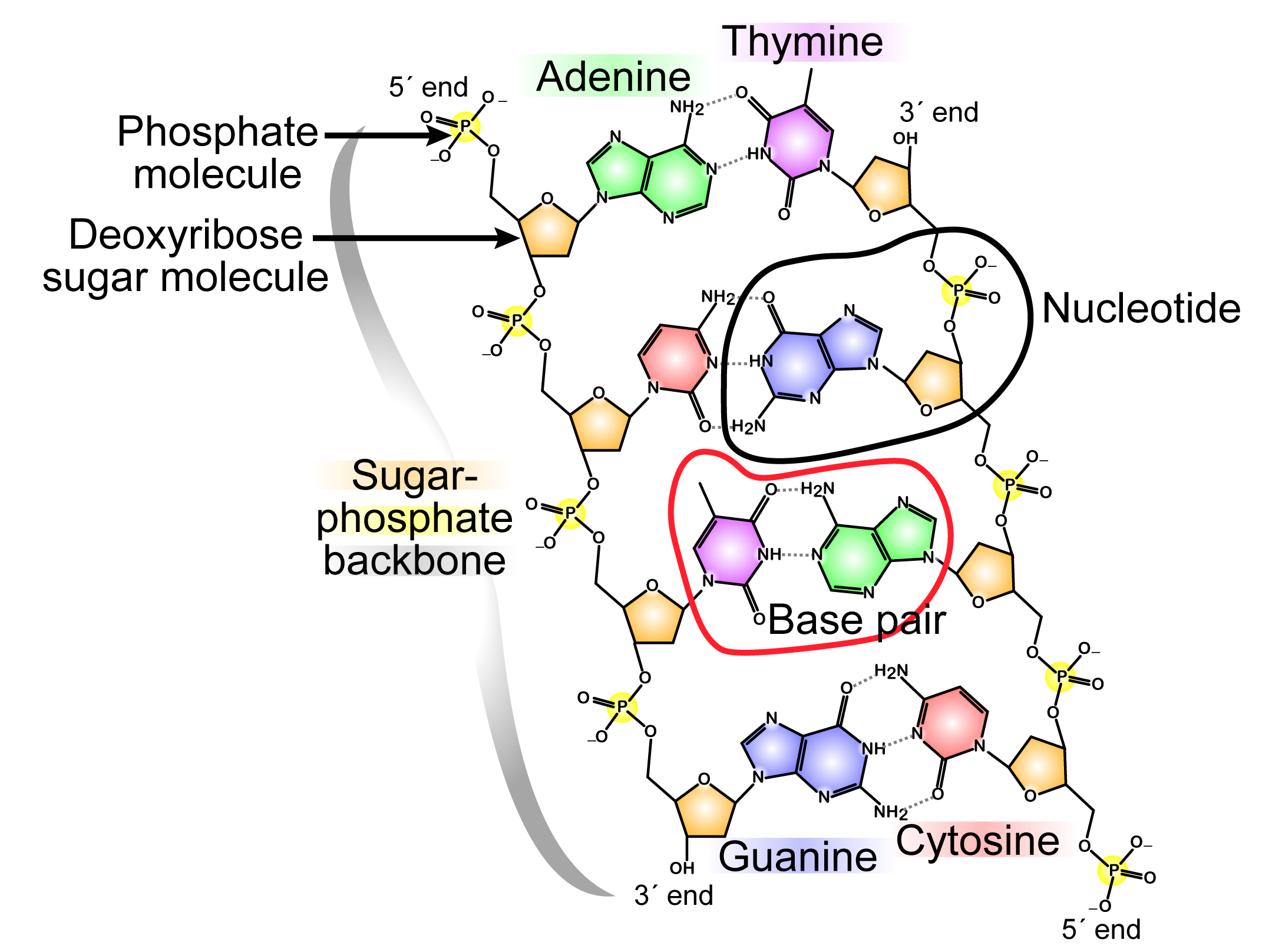
Chemical structure of a strand of DNA showing the nucleic acids (adenine, cytosine, guanine, and thymine), nucleotides (nucleic acid and a piece of the backbone), and base pairs. Illustration by Madprime (Wikimedia Commons, CC0 1.0 Universal/Public Domain Dedication).
The specific order of these nucleotides, known as the DNA sequence, is unique to each biological organism. DNA is often referred to as the “blueprint” of life, as it contains all the instructions necessary to form and maintain the organism. Most organisms are composed of sequences that are billions of bases long. These sequences make up one or more chromosomes.
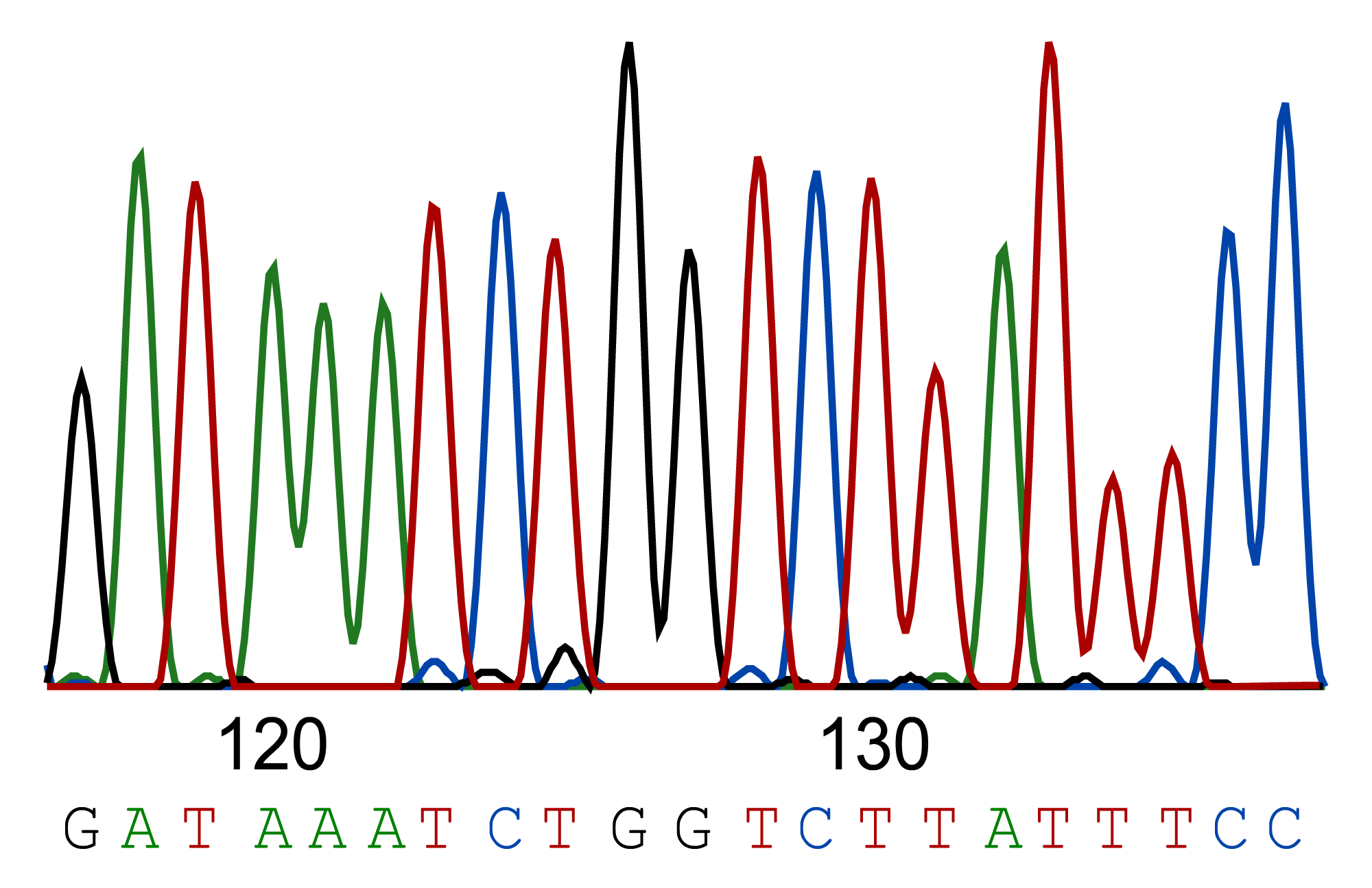
Illustration showing the results of DNA sequencing. The nucleic acids are represented by different-colored peaks in a chromatogram and as letters. Illustration by Sjef (Wikimedia Commons, public domain).
Some segments of the nucleotide sequence that make up a chromosome are genes. Other parts of the sequence have functions such as maintaining the structure of the chromosomes, regulating gene expression, etc. Some segments have an unknown function or may have no function at all.
A popular misconception is that the complexity of an organism is related to the size of its genome. In reality, the number of genes and size of the genome of an organism are not related to its complexity. For example, maize and rice are very similar plants, and yet the genome size of maize is much larger. Rice has a genome size of up to 430,000,000 base pairs, whereas maize has a genome size of about 2,400,000,000 base pairs. By comparison, a human genome is about 3,100,000,000 base pairs. Bread wheat (Triticum aestivum) has about 17,000,000,000 base pairs! Bread wheat has such a large genome because it has inherited genomes from three different ancestral species of plant.
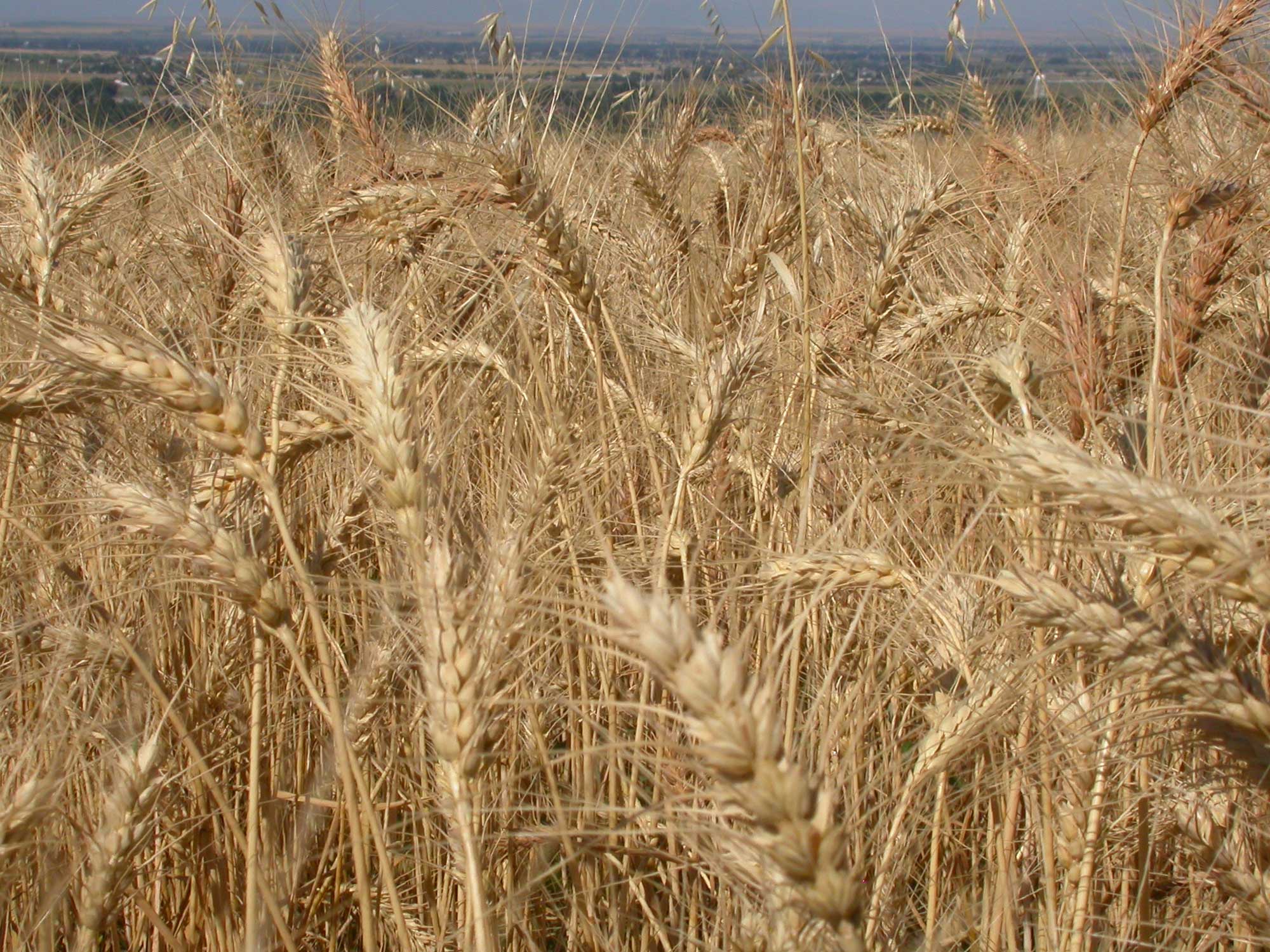
A field of bread wheat (Triticum aestivum) in Montana, U.S.A. "Triticum aestivum," Photo by Matt Lavin (flickr, Creative Commons Attribution-ShareAlike 2.0 Generic license, image resized).
Sexual reproduction and variation
Sexual reproduction allows for fantastic variation within a species, and contributes greatly to a species’ resilience to changes in its environment, disease, and other deleterious variables. Sexual reproduction generates variation within populations of organisms by shuffling the genetic deck between generations.
Meiosis
In organisms with sexual reproduction, chromosomes are passed down from generation to generation via gametes. A gamete is a reproductive cell with just one set of chromosomes. In both humans and plants, the gametes are called sperm (the male gamete) and egg (the female gamete).
In humans, gametes are formed by a process called meiosis. Meiosis is a type of cell division in which a single diploid cell (a cell with two sets of chromosomes) divides twice to produce four haploid daughter cells (cells each having one set of chromosomes). Furthermore, each of the four daughter cells produced by meiosis are genetically different from one another. In humans, each somatic cell (meaning, each non-reproductive cell or cell that is not a gamete) has 46 chromosomes, and each gamete has 23 chromosomes. Human cells with 46 chromosomes are diploid, and human cells with 23 chromosomes are haploid.
Grasses, like humans and all flowering plants, are diploid. However, the flowering plant life cycle is more complicated than the human life cycle. In flowering plants, meiosis does not produce gametes directly. Rather, meiosis produces cells called spores. The spores grow into tiny structures (the pollen grain and the embryo sac in the ovule) that contain the gametes. In maize, for example, most cells have 20 chromosomes, but spores, gametes, and some other cells in the reproductive structures (pollen grain and embryo sac) have 10 chromosomes. Maize cells with 20 chromosomes are diploid, and maize cells with 10 chromosomes are haploid.
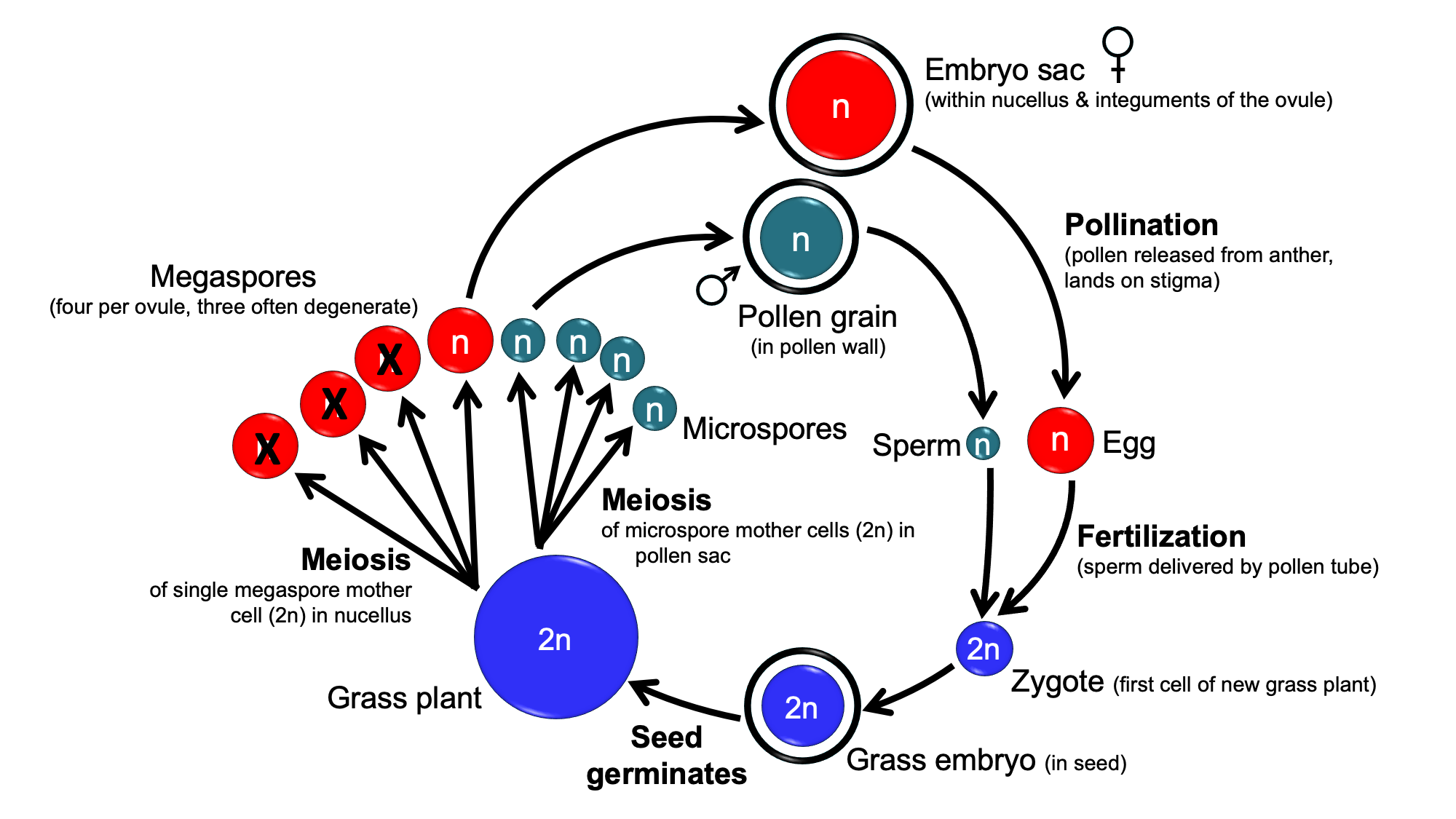
Grass life cycle diagram. Diagram by Elizabeth J. Hermsen, adapted from a diagram on Digital Encyclopedia of Ancient Life.
Meiosis I
Meiosis consists of two broad stages: Meiosis I and Meiosis II. Meiosis I is the first division of meiosis. At the beginning of meiosis, the chromosomes have duplicated. Thus, each chromosome consists of two chromatids (DNA strands) joined at a region called a centromere.
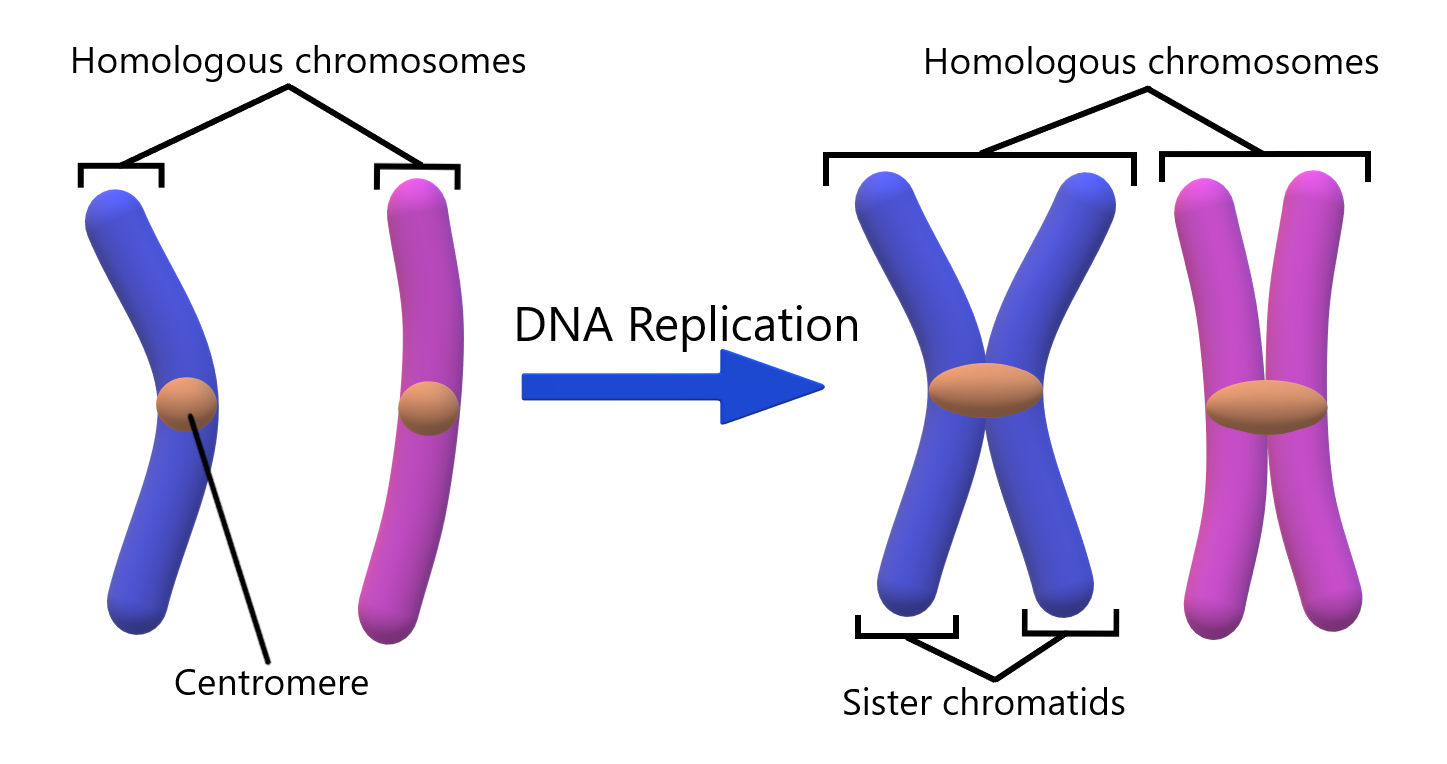
Diagrams showing chromosome duplication and pairs of homologous chromosomes. Diagram by Christinelmiller (Wikimedia Commons, Creative Commons Attribution-ShareAlike 4.0 International license).
The homologous chromosomes line up, meaning that the set of chromosomes from each parent lines up with its counterpart. For example, maternal Chromosome 1 (meaning, the copy of Chromosome 1 inherited from the mother) lines up with paternal Chromosome 1 (meaning, the copy of Chromosome 1 inherited from the father), and so on. The chromosomes also pair such that the respective alleles on each chromosome are paired. For example, Gene A on maternal Chromosome 15 will pair with Gene A on paternal Chromosome 15, and so on.
Once the chromosomes are paired, segments of matched chromosomes are shuffled between them in an event known as crossing over or recombination. During this process, the DNA is broken apart and rejoined. Crossing over results in a pair of chromosomes that are each a mixture of the DNA inherited from the individual's mother and father. Segments of DNA are usually moved intact, meaning that linked segments (such as whole genes or regulatory sequences of the genome) are moved together. This helps to prevent the inheritance of non-functional gene fragments.
Once these ‘new’ chromosomes are formed, the two sets of chromosomes separate and the cell that they are in divides, forming two daughter cells. Each of the two cells formed by cell division in meiosis I is haploid, or has only has one set of chromosomes. Each chromosome still consists of two chromatids. The daughter cells are genetically different from one another.
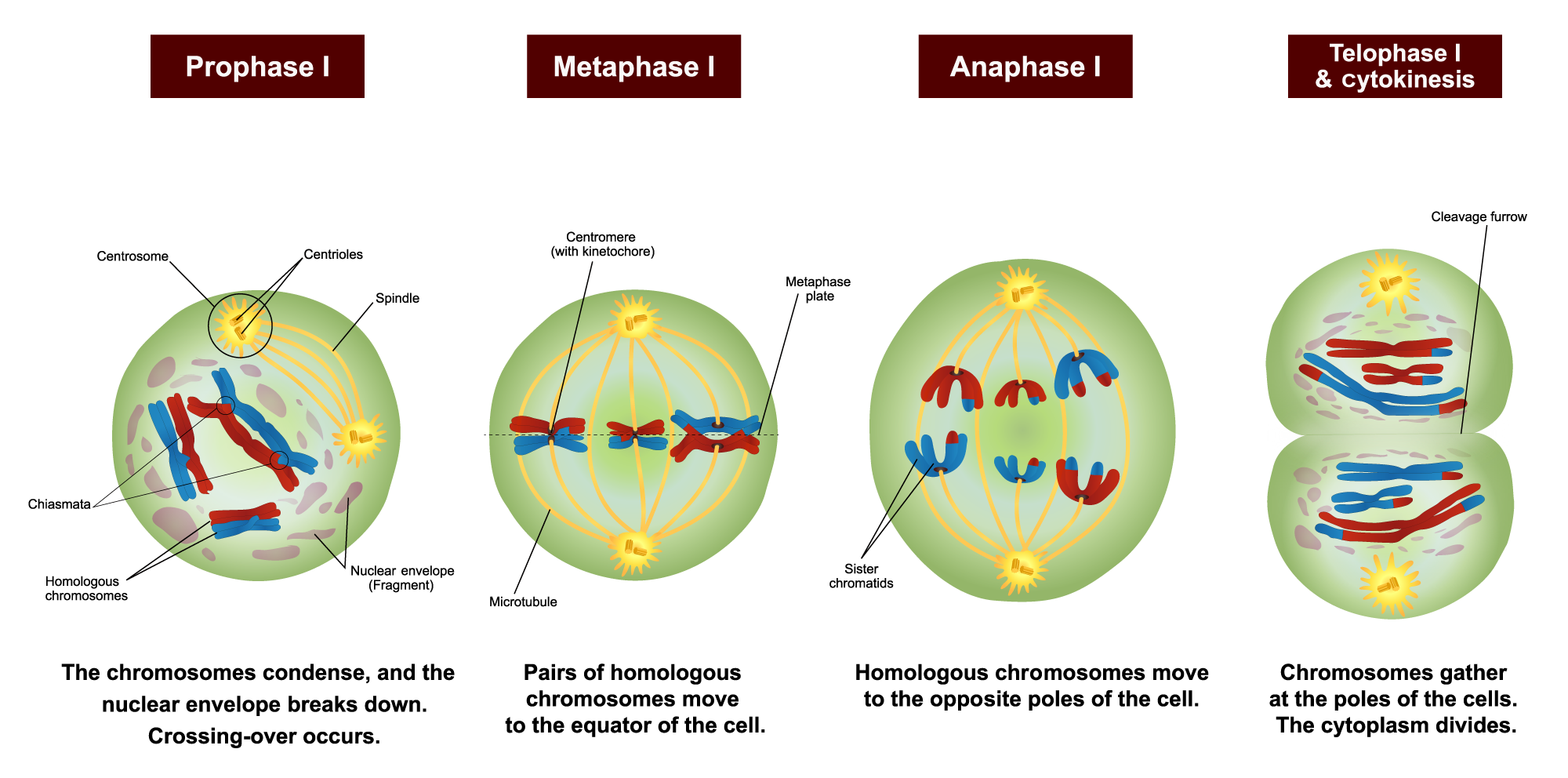
Meiosis I (first division). In the first part of meiosis, chromosomes duplicate so that each chromosome has two chromatids (strands) joined at a central point called the centromere. Recombination, the swapping of DNA between homologous chromosomes. Homologous pairs of chromosomes then separate, with one member of each pair ending up in each daughter cell. Thus, each of the two daughter cells has one set of chromosomes, each made up of two chromatids joined at a centromere. Diagram by Ali Zifan (Wikimedia Commons, Creative Commons Attribution-ShareAlike 4.0 International license, image cropped and resized).
Meiosis II
Meiosis II is the second division of meiosis. In meiosis II, the two chromatids making up each chromosome in each of the daughter cells separate, and two more haploid daughter cells are formed by cell division. Because of the recombination that occurred during meiosis I, the two chromatids on each chromosome are not identical. Thus, at the end of meiosis II, four haploid cells with different genotypes are created. In humans, these cells are gametes; in plants, these cells are spores.
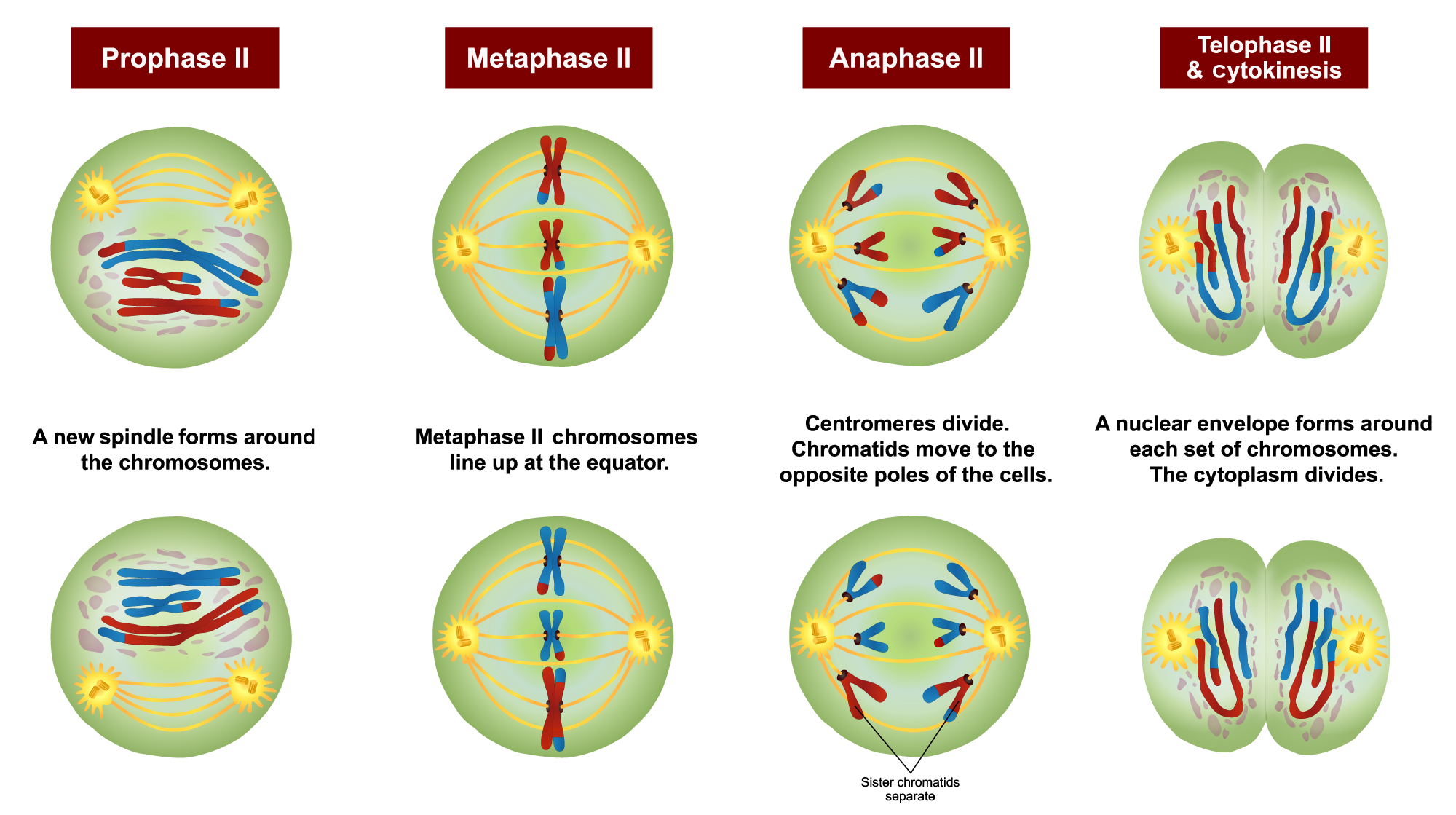
Meiosis II (second division). In the second part of meiosis, the duplicated strands of each chromosome separate, so that each daughter cell receives one of the sister chromatids. Once the chromatids separate, each is considered a separate, single-stranded chromosome. Each of the four cells that are formed at the end of meiosis II is genetically unique. Diagram by Ali Zifan (Wikimedia Commons, Creative Commons Attribution-ShareAlike 4.0 International license, image cropped and resized).
Fertilization
Fertilization occurs when gametes fuse to form one cell called a zygote that has two sets of chromosomes. The zygote is the first cell of a new individual. The zygote begins to develop into an embryo. The embryo has its own unique set of genes, one contributed be each parent (in other words, one is contributed by the sperm and one by the egg).
Laws of Inheritance
Excluding reproductive cells (like gametes and spores) and haploid structures (like pollen grains and embryo sacs), each individual cell in a diploid organism, like a human being or a maize plant, has one set of chromosomes from each parent. Each individual cell therefore has two copies of each chromosome and thus two copies of each gene or DNA segment. Often, these genes come in two or more different forms called alleles. Alleles help to determine the physical traits of an individual organism and account for variation among the traits of organisms within a population.
With some exceptions, the inheritance of alleles follows the Laws of Inheritance that were devised by Gregor Mendel in 1866 based on the results of his famous experiments on pea plants. They describe how alleles are inherited. Notably, while these laws are generally true, there are exceptions and complications, some of which are described below.
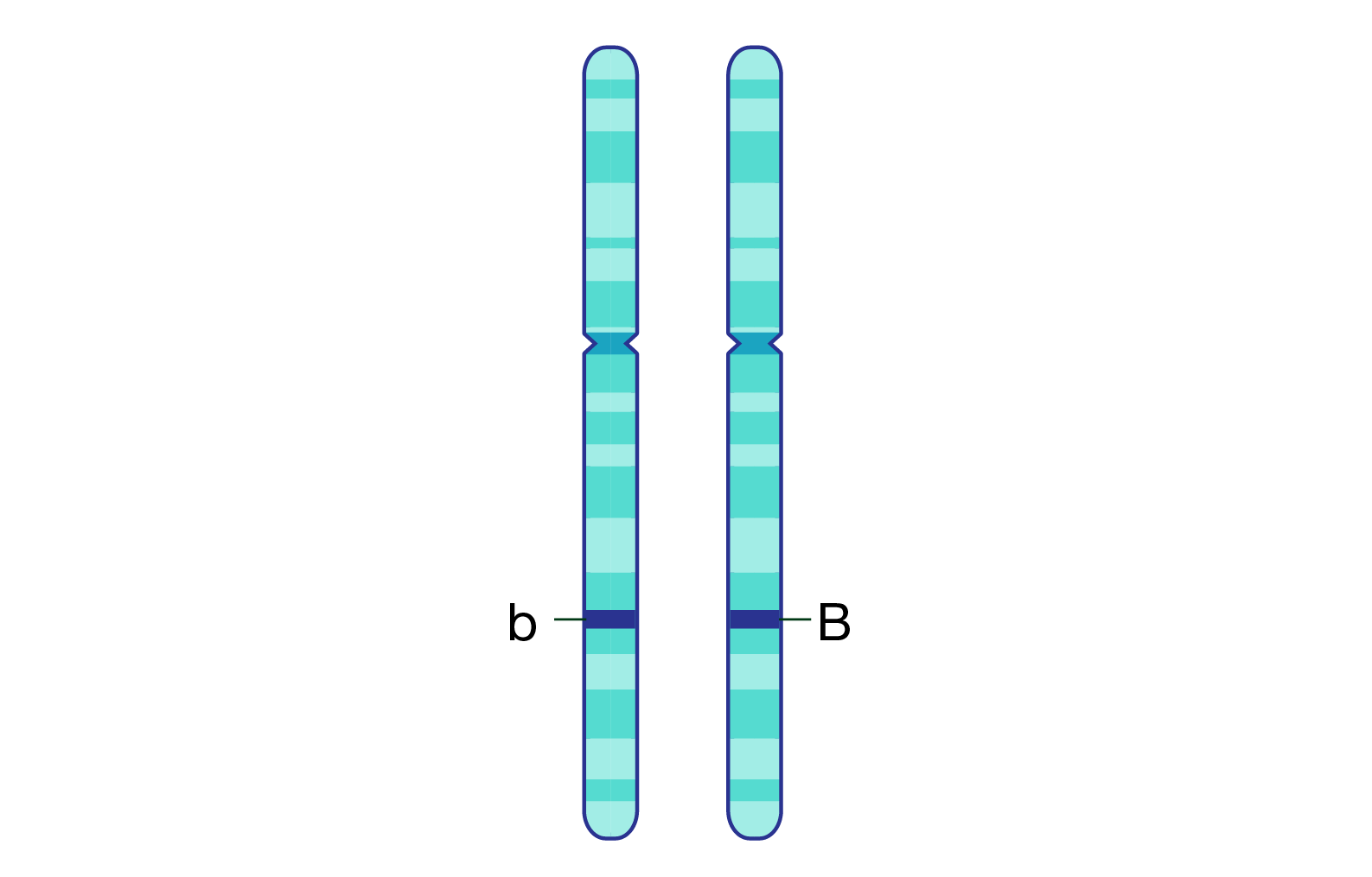
Diagram illustrating alleles (variations) of a gene on two homologous (equivalent) chromosomes. Illustration courtesy of National Human Genome Research Institute (public domain).
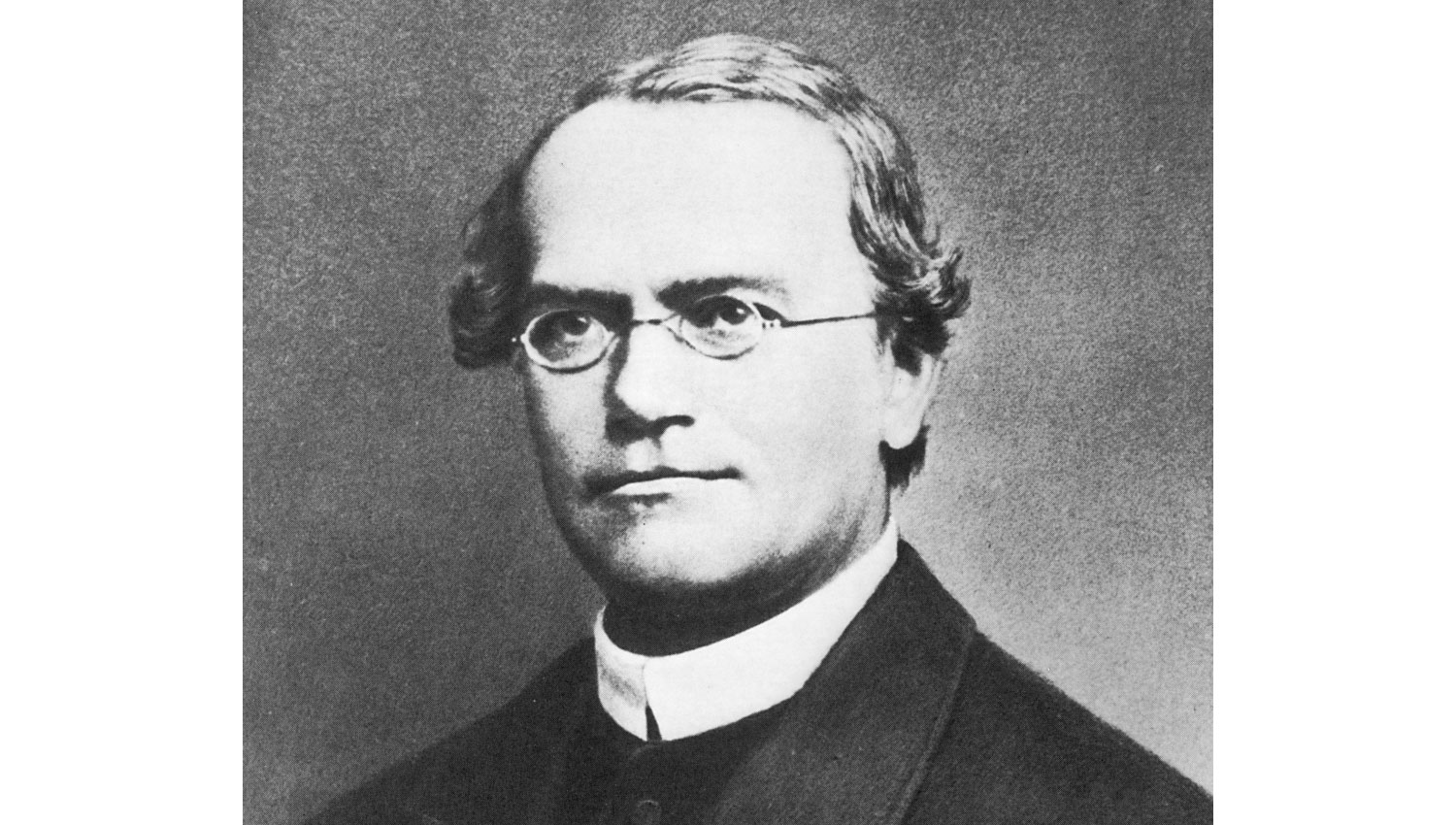
Gregor Mendel (1822-1884). Source: Author unknown, Wikimedia Commons (public domain).
Law of Segregation
The Law of Segregation states that each gamete or spore receives only one of the two possible copies (the paternal copy or the maternal copy) of each chromosome from a diploid individual. In other words, each pair of chromosomes from a diploid individual separates into different cells. Segregation of chromosomes happens as a result of meiosis.
Law of Independent Assortment
The Law of Independent Assortment states that different traits are inherited independently of one another. All the maternal alleles, or all the paternal alleles, do not end up in the same gamete or spore, but rather the maternal and paternal alleles assort (separate into different cells) independently of each other during meiosis.
The exception to the Law of Independent Assortment is linkage. When two genes or DNA segments are located near each other on the same chromosome, they are more likely to be inherited together. Linked genes can be shuffled by recombination. Linkage and recombination are very useful for scientists who are trying to map genes, or determine the chromosomal location of specific genes or DNA segments; there is a direct statistical relationship between how far apart DNA segments are and how often they recombine.
Alleles for various phenotypes are sometimes misaligned, and genes and segments can be broken apart in the process. Although misalignment and breakage is relatively rare, one can imagine that this type of error can result in inheritance of, for instance, two copies of one allele or missing alleles, which could be lethal. On the other hand, this phenomenon could result in new phenotypes that may be advantageous to the organism.
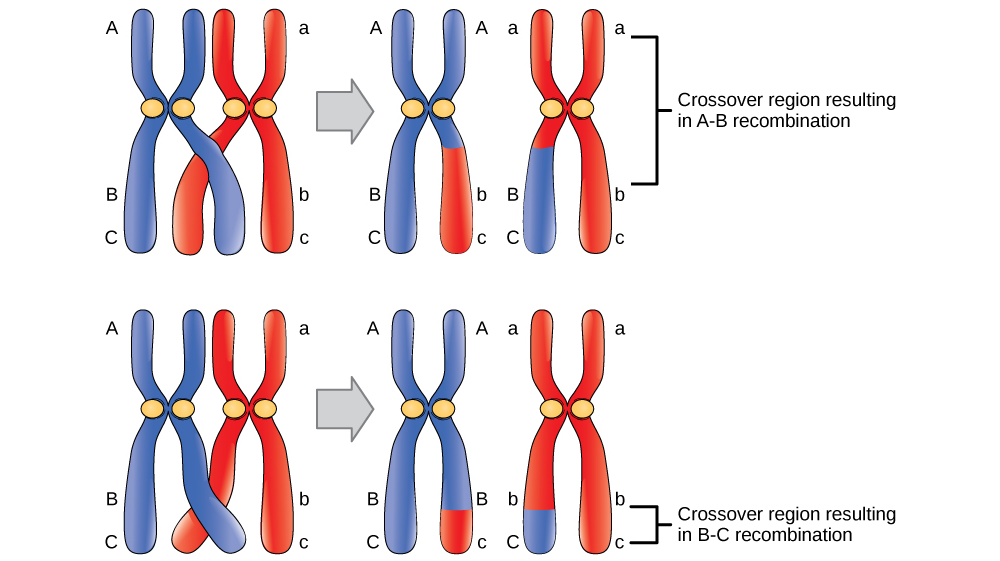
Original caption: "Crossover may occur at different locations on the chromosome. Recombination between genes A and B is more frequent than recombination between genes B and C because genes A and B are farther apart; a crossover is therefore more likely to occur between them." Source: CNX OpenStax Biology figure 17.11 via Wikimedia Commons (Creative Commons Attribution 4.0 International License).
Law of Dominance
The Law of Dominance states that the presence of an allele does not mean that its trait will be expressed. If an individual inherits the same allele from both parents, that individual is homozygous for that gene (“homo” = same). If an individual inherits a different allele from each parent, that individual is heterozygous for that gene (“hetero” = different). If two alleles of an inherited pair are different, one may mask the effects of the other. In such a case, the dominant allele determines the organism’s appearance, while the recessive allele has no noticeable effect.
In Mendel's experiments on peas, the gene controlling flower color had dominant and recessive alleles; pink was dominant, white was recessive. Thus, if a pea inherited the allele for pink flowers, it would have pink flowers, regardless of whether it also had an allele for white flowers or not. It would only have white flowers if it inherited two recessive alleles for white flowers.
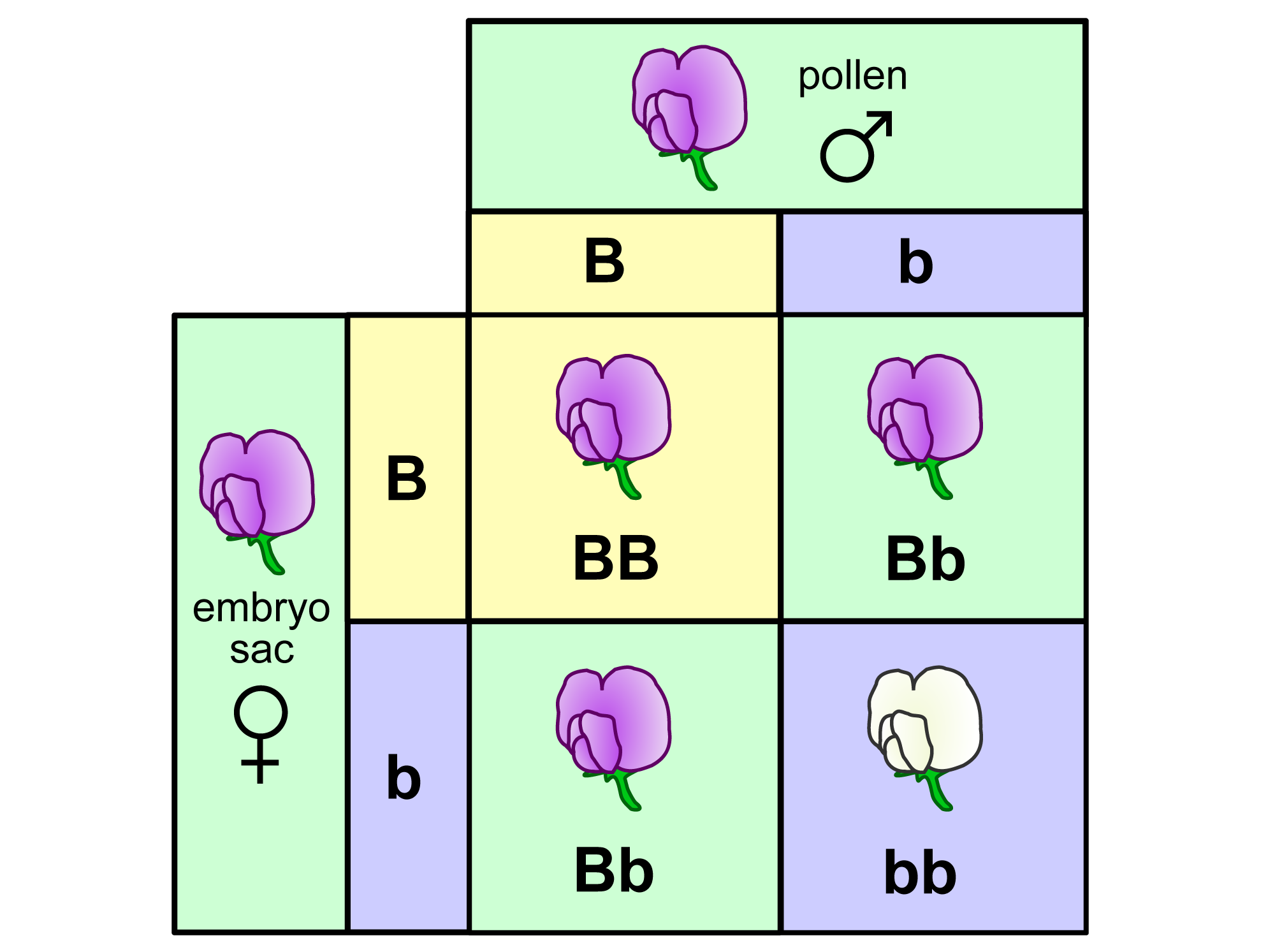
An example of inheritance of dominant and recessive alleles in peas, and the effects on the phenotypes of the flowers. Each parent plant is heterozygous for flower color, with one dominant allele for pink flowers (B) and one recessive allele for white flowers (b). Flower color in the offspring is based on whether each inherits at least one dominant allele (pink flowers) or two recessive alleles (white flowers). Figure by Madprime (Wikimedia Commons, CC0 1.0/public domain dedication).
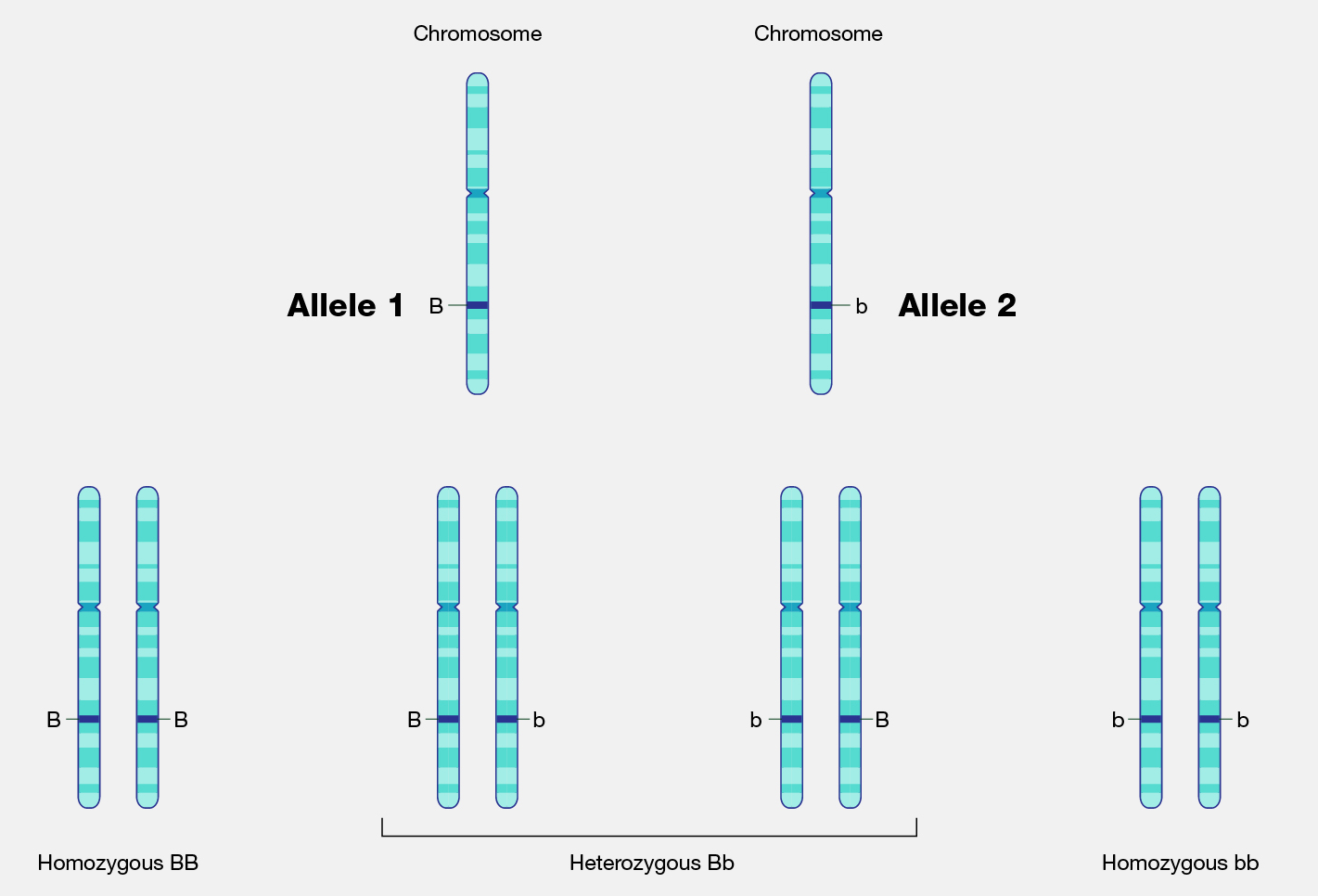
Diagrams illustrating alleles (variations) of a gene on a chromosome. The top row shows the two alleles, B (allele 1) and b (Allele 2) in the same position on homologous (equivalent) chromosomes. The bottom row shows the four possible patterns of inheritance these alleles in a diploid organism: BB, Bb, and bb. Illustration courtesy of National Human Genome Research Institute (public domain).
Often, inheritance is more complex than a simple dominant-recessive relationship. Pairs or groups of genes may together determine the expression of a single trait, with none being fully dominant or fully masked. In humans, for example, there are many possible alleles for the genes that determine eye color, resulting in eyes that are blue, brown, green, hazel, and other variations. For whole organisms, there are usually tens of thousands of genes working together to create the appearance and behavior of an organism.
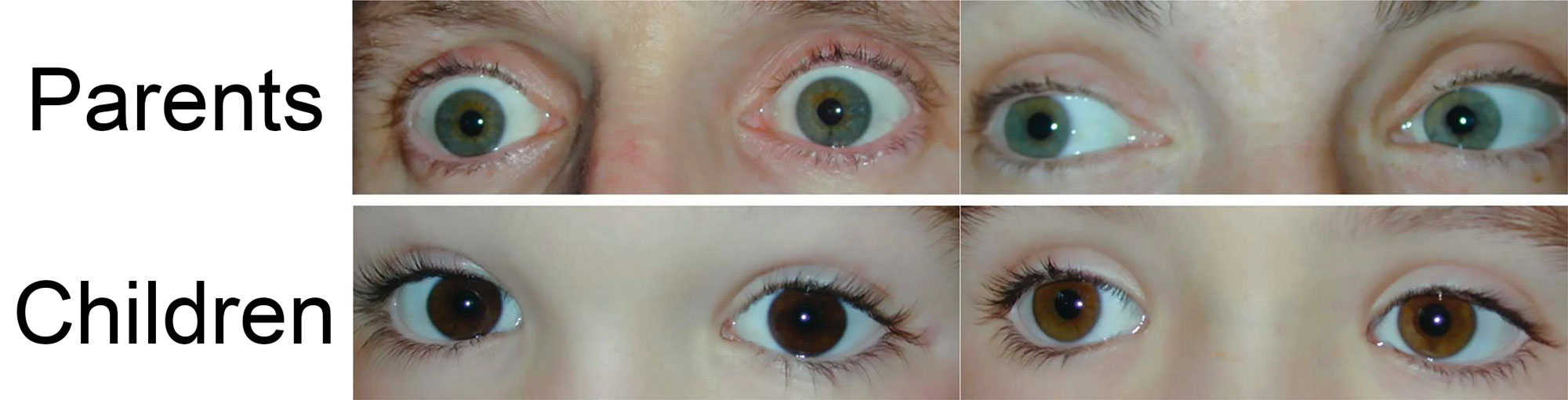
Examples of eye color in a pair of human parents and their children. While blue or green eyes are often considered recessive, the genetics of eye pigmentation in humans and, thus, the inheritance of eye color are complex. In this case, two parents with blue-green eyes have two children with brown eyes, possibly because the genes controlling light eye pigmentation are different in each parent. Source: Modified from figure 6 in Mackey (2022) Eye 36: 704-715 (Creative Commons Attribution 4.0 International license).
Other sources of variation
Mutations
Mutations are changes to one or more nucleotides in a DNA sequence. They can be caused by mutagens such as radiation (including ultraviolet radiation from the sun) or chemicals, or simply by mistakes that occur during DNA replication. Mutations in somatic cells (nonreproductive cells) can cause problems, such as cancer, in an organism but are not heritable. Mutations present in reproductive cells (gametes) are inherited by the progeny.
Most of an organism’s DNA sequence is not actually translated into genes and is called non-coding DNA. Some of this non-coding DNA is involved in maintaining the structure of the DNA, as well as in gene expression and regulation. If a mutation occurs in a non-coding region, it may not have any effect on the phenotype of the organism.
Even within a gene, some mutations may not lead to a change in the organism. These are called neutral mutations. One reason that mutations may be neutral is because of the way that they DNA code works. Each set of three DNA bases in a gene codes for an amino acid. Amino acids are the building blocks of proteins. There are a total of 20 amino acids, and most can be coded for by more than one three-letter combination of bases. Because of this, a change in a single base will not necessarily result in a change in the amino acid that a segment of a DNA sequence codes for.
If a mutation does cause some change in an organism, it is called a functional mutation. A mutation, sometimes even just a single nucleotide change, can cause a gene to no longer function, no longer encode for the same protein, or create some new function. Most of these changes are detrimental, or even lethal, to an organism. If they are lethal, the organism most likely would not have a chance to reproduce, and, therefore, that mutation will not be passed on.
Sometimes, however, a mutation can have effects beneficial to the organism just by chance. Suppose a change in a gene made an organism more resistant to a disease or better able to tolerate drought. That organism would be more likely to survive and pass on the new genes to more progeny. This is how species are constantly changing and adapting to their environment.
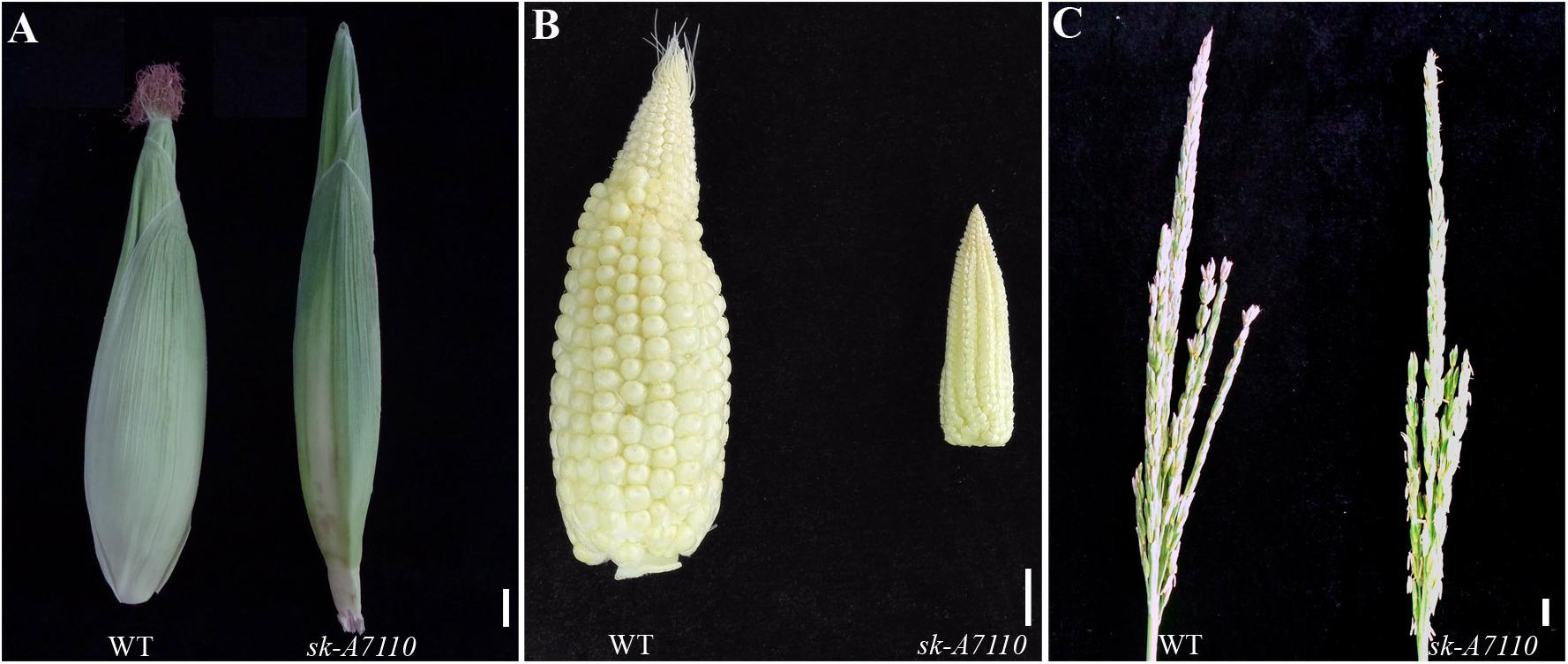
Comparison of phenotypes between typical or wild-type maize (WT) and the sk-A7110 maize mutant. The insertion of one additional nucleotide on chromosome 2 of the sk-A7110 maize mutant results in the development of an ear without silk (A), pistils that break down and produce a smaller ear (B), and tassels with fewer branches (C). This is an example of a detrimental functional mutation. Source: Figure 1 from Zhao et al. (2018) Frontiers in Plant Science 9:1227 (Creative Commons Attribution 4.0 International license).
Transposition
Transposition is a phenomenon where genes or small pieces of DNA move to different locations in the genome, sometimes causing mutations. These mobile segments of DNA, formerly called “jumping genes,” are now called mobile genetic elements or transposons. Transposition can promote genetic variation but can also cause diseases.
In maize, transposition can affect kernel color. The pigment of each maize kernel is found in the outer layer, called the aleurone layer, of the endosperm. Endosperm is a specialized food tissue that is formed as a product of double fertilization in flowering plants. Thus, the endosperm is genetically different in each maize kernel, and the combination of genes and alleles that control kernel pigmentation may vary from kernel to kernel on the same cob, depending on the genetics of the gametes contributed by each of their parent plants.
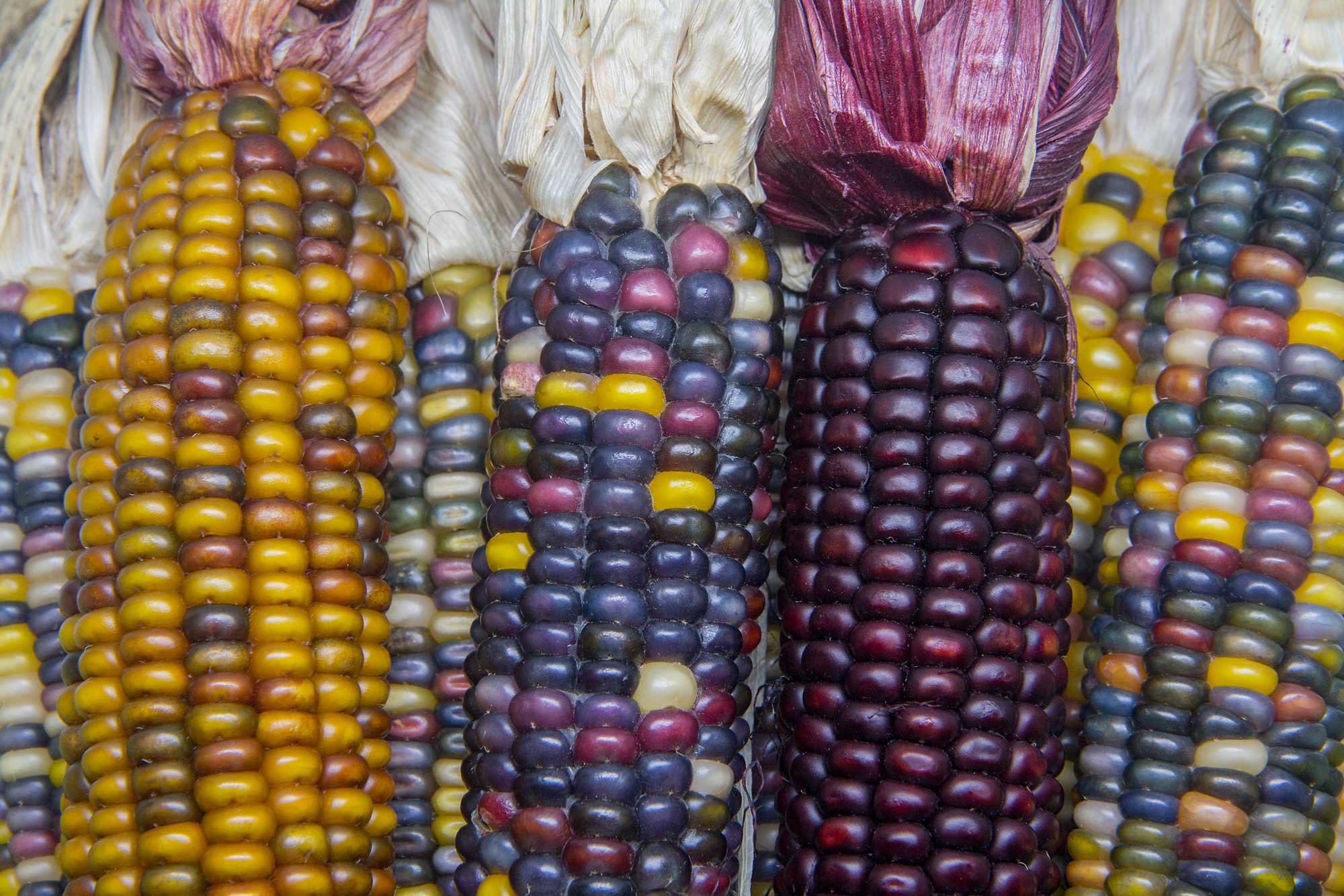
Multicolored kernels of corn. Photo by Sally Robertson (Colored Pencil magazine on flickr, Creative Commons Attribution 2.0 Generic license, image resized).
Barbara McClintock discovered transposition while studying maize kernel color. She hypothesized that the presence of a "jumping gene" was responsible for striped or speckled patterns in some maize kernels. In these kernels, a gene "jumped" or changed position in some cells but not others, affecting the expression of genes that control pigmentation. Thus, some cells had color whereas others were colorless (appearing white or yellow), making the patterns on striped and speckled kernels.
This discovery was so unique that many scientists did not believe her work for many years. She finally won a Nobel Prize for this discovery years later in 1983, when she was 81 years old. She remains the only woman to receive an unshared Nobel Prize in the category of “Medicine or Physiology.”
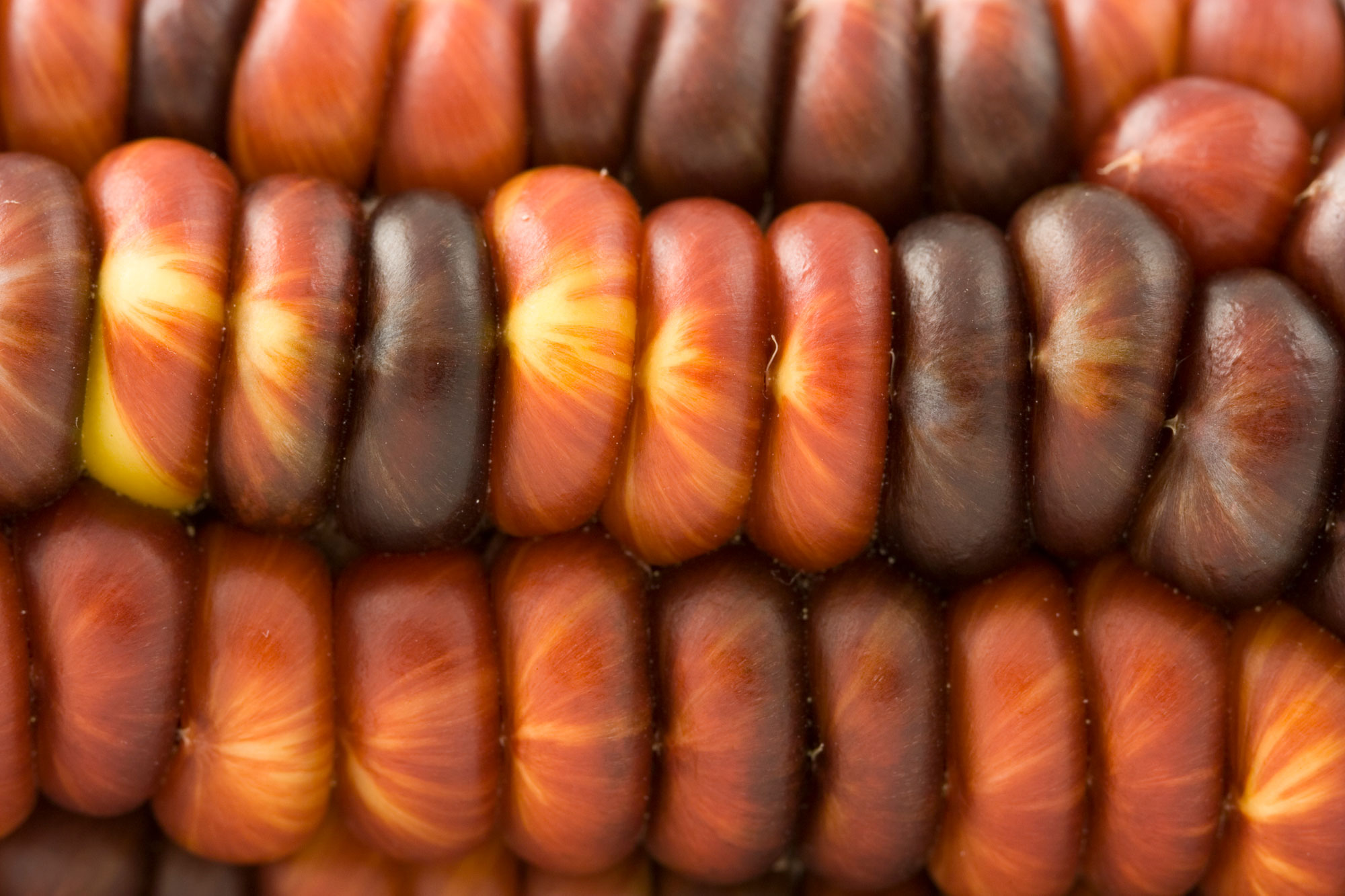
Striped kernels of corn. Photo by Petr Kratochvil (Wikimedia Commons, Creative Commons Attribution 3.0 Unported license, image resized).
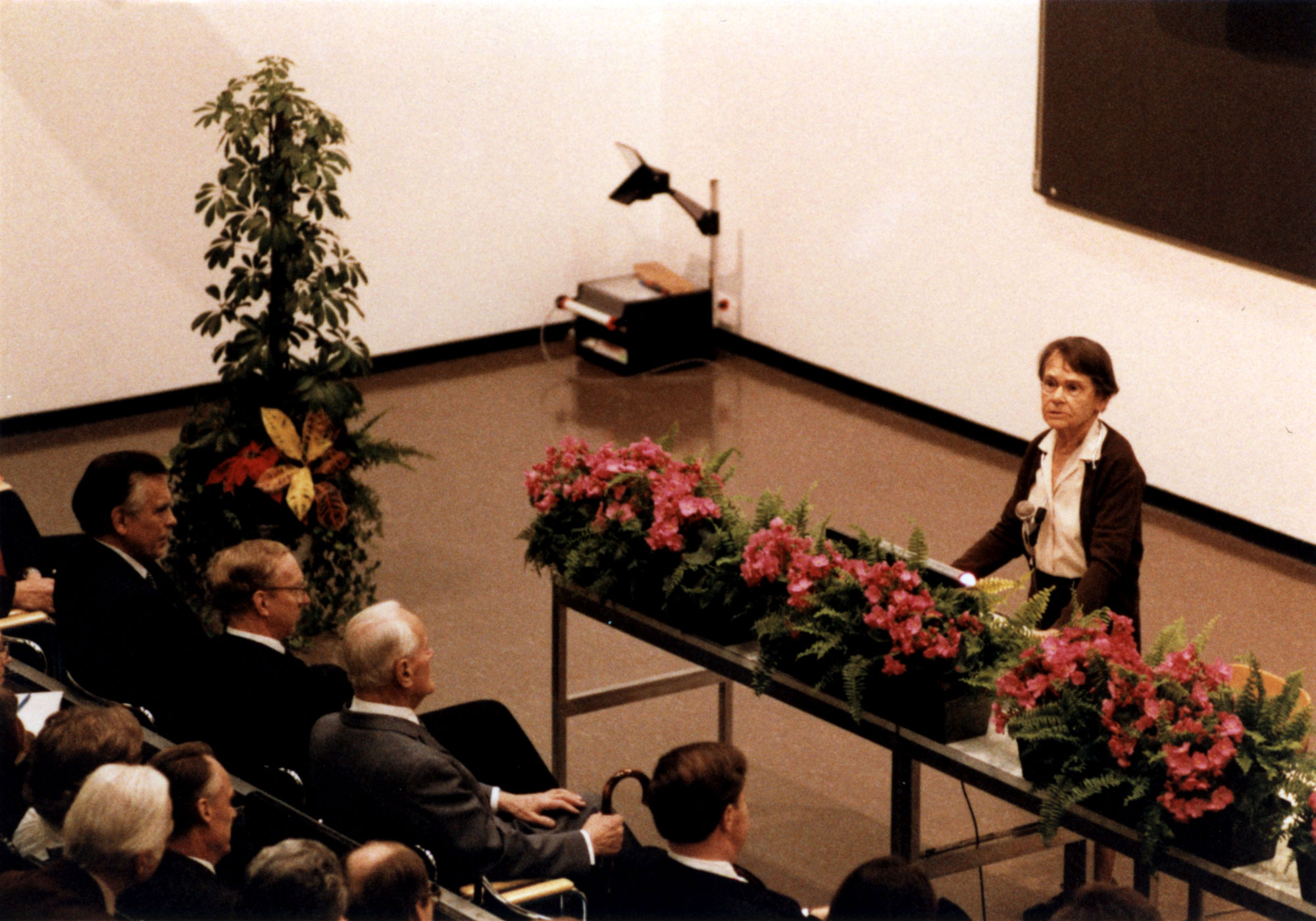
Barbara McClintock giving her Nobel lecture, 1983. Photo author unknown, National Institutes of Health via Wikimedia Commons.
Polyploidy
Ploidy refers to the number of complete sets of chromosomes in each cell in an organism. Humans are diploid organisms; each person has 2 sets of 23 chromosomes, one from each parent in every somatic cell, or non-reproductive cell, for a total of 46 chromosomes. Among domesticated grasses, maize and sorghum are examples of diploid plants.
Some domesticated grasses are polyploid. Polyploid organisms have more than two sets of chromosomes per cell. Polyploids are often larger and have larger fruits or higher seed yields than their diploid counterparts. Polyploids can arise naturally or may be human-created. Some examples of domesticated plants of various ploidy levels are:
- Diploid (2x): two sets of chromosomes per cell. Examples: maize (Zea mays), tomato (Solanum lycopersicum), rice (Oryza sativa).
- Triploid (3x): three sets of chromosomes per cell. Examples: seedless banana (Musa x paradisiaca), seedless watermelon (Citrullus lanatus).
- Tetraploid (4x): four sets of chromosomes per cell. Examples: durum wheat (Triticum durum), some types of cotton (Gossipyium barbadense, G. hirsutum), potato (Solanum tuberosum).
- Hexaploid (6x): six sets of chromosomes per cell. Examples: bread wheat (Triticum aestivum), kiwifruit (Actinidia deliciosa).
- Octoploid (8x): eight sets of chromosomes per cell. Examples: strawberry (Fragaria x ananassa), some types of sugarcane (Saccharum officinarum).
There are two main types of polyploidy in plants. An autopolyploid is a polyploid in which a set of chromosomes from the same species is multiplied in an individual. Octoploid sugar cane (Saccharum officinarum) is an autopolyploid, so it has eight sets of chromosomes, all from the same parent species. An allopolyploid is a polyploid in which diploid sets of chromosomes from individuals in different species are multiplied. Thus, allopolyploids are hybrids or have hybrid origins. Polyploid durum and bread wheats, for example, are allopolyploids of species of goat grass (Aegilops) and wheat (Triticum).
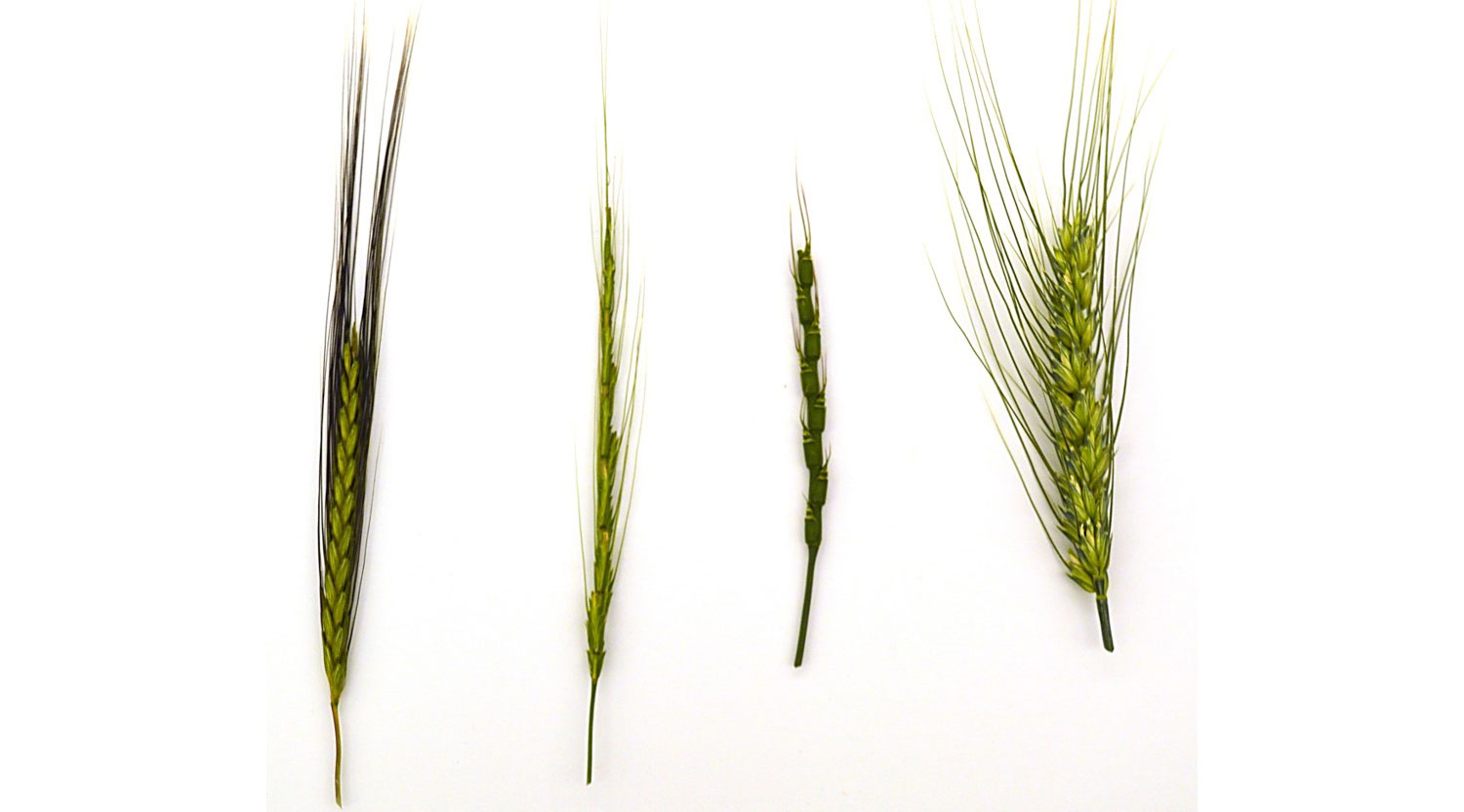
Bread wheat (an allopolyploid) and its ancestors. From left to right: Red wild einkorn wheat (Triticum urartu), goatgrass (Aegilops speltoides var. ligustica), Tausch's goatgrass (Aegilops tauschii), and bread wheat (Triticum aestivum). Photo by Pat Byrne (from G. M. Volk and P. F. Byrne, Crop wild relatives and their use in plant breeding. Colorado State University, eBook, public domain).
Note that when discussing sexual reproduction in polyploids, gametes of polyploids have half the number of sets of chromosomes as the parent plants. Thus, the gamete of a tetraploid plant like bread wheat has two sets of chromosomes, whereas the gamete of an octoploid plant like sugar cane has four sets of chromosomes. This can be a bit confusing, because the use of "diploid" in the context of discussing a plant's life cycle and "diploid" in the context of discussing ploidy in plant genetics are not exactly the same things.
Sometimes, the notation "n" is used to indicate the number of chromosomes in a gamete, whereas the notation "x" is used to indicate the number of chromosomes in one complete set of chromosomes in a given organism. In a diploid plant, n and x are the same; in a polyploid plant, n and x are different. Thus, in diploid maize, one set of chromosomes is 10 chromosomes (x = 10), the gametes of maize have 10 chromosomes (n = 10), and maize plants have 20 chromosomes (2x = 20, 2n = 20). In octoploid sugar cane, however, a single set of chromosomes consists of 10 chromosomes (x = 10), a sugar cane gamete has 40 chromosomes (n = 40), and a sugar cane plant has 80 chromosomes (8x = 80, 2n = 80).
Chromosome numbers in most polyploid plants are in multiples of two. This is because meiosis cannot occur properly with an odd number of sets of chromosomes. In a triploid plant, two of the sets of chromosomes will be able to pair up during meiosis, whereas one set of chromosomes will not. Notably, triploid watermelon (Citrullus lanatus) and triploid bananas (Musa x paradisiaca) are seedless and parthenocarpic, meaning that they can produce fruit without fertilization. Triploid bananas are propagated asexually. Triploid watermelons are produced by crossing diploid and tetraploid watermelons to produce seeds from which triploid plants are grown; thus, the gamete from the diploid plant contributes one set of chromosomes and the gamete from the tetraploid plant contributes two sets.
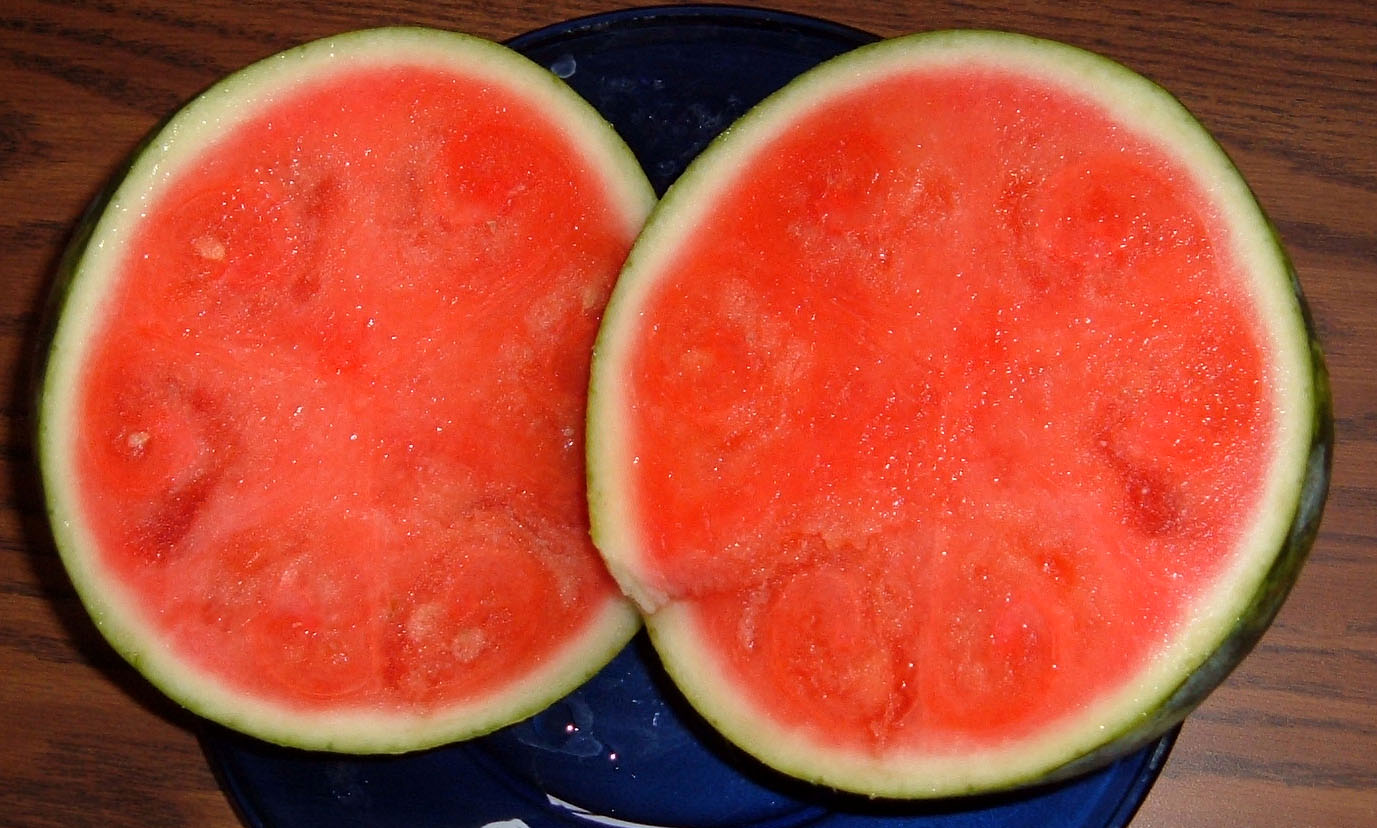
Seedless watermelon. Photo by Scott Ehardt (Wikimedia Commons, public domain).
Resources
Websites
Educational Resources, National Human Genome Research Institute: https://www.genome.gov/About-Genomics/Educational-Resources
Books, articles, and reports
Bertrom, G. 2021. The 'jumping genes' of maize. Basic Cell and Molecular Biology. LibreTexts, University of Wisconsin-Milwaukee. https://bio.libretexts.org/Bookshelves/Cell_and_Molecular_Biology/Book%3A_Basic_Cell_and_Molecular_Biology_(Bergtrom)
Burr, B., and F. Burr. 2000. How do seedless fruits arise and how are they propagated? Scientific American, October 2, 2000. https://www.scientificamerican.com/article/how-do-seedless-fruits-ar/
Evert R. F., and S. E. Eichhorn. 2013. Raven Biology of Plants, 8th ed. W.H. Freeman and Co., New York, New York.
Mackey, D. A. 2022. What colour are your eyes? Teaching genetics of eye colour & colour vision. Edridge Green Lecture RCOpthth Annual Congress Glasgow May 2019. Eye 36: 704-715. https://doi.org/10.1038/s41433-021-01749-x
Nickle, T., and I. Barrette-Ng. 2020. Polyploidy arises from changes in whole sets of chromosomes. Introduction to Genetics. LibreTexts. https://bio.libretexts.org/Courses/City_College_of_San_Francisco/Introduction_to_Genetics/02:_Chromosomes_Mitosis_and_Meiosis/2.05:_Polyploidy_Arises_from_Changes_in_Whole_Sets_of_Chromosomes